I have noticed, too … that the soil of Egypt does not resemble that of the neighbouring country of Arabia, or of Libya, or even of Syria … but is black and friable as one would expect of an alluvial soil formed of the silt brought down by the river from Ethiopia. The soil of Libya is, as we know, reddish and sandy, while in Arabia and Syria it has a larger proportion of stone and clay.
4.1 Introduction
This chapter provides a brief outline of the geology, geomorphology and soils of the Nile Basin. We pay special attention to the scope and limitations of using evidence from Quaternary geology, landforms and soils to reconstruct prehistoric environments and changes in local and regional climate. Table 4.1 is a summary of the types of evidence from the Nile Basin that have been used to reconstruct prehistoric environments as well as to determine past fluctuations in Nile discharge and sediment transport and deposition. Williams (Reference Williams2014, chapters 7 to 17) provides a detailed evaluation of each of the types of evidence listed in Table 4.1.
Table 4.1 Evidence used to reconstruct prehistoric environments in the Nile Basin
Adapted from Williams (Reference Williams2014).
Implicit in this table is that the age of each proxy needs to be determined by at least one and preferably by two or more independent dating techniques. Williams (Reference Williams2014, chapter 6) provides a review of dating methods. The most common dating techniques relevant to the present volume are radiocarbon (14C) dating for organic samples younger than about 40–50,000 years; luminescence dating for sediments that contain quartz or feldspar, with a time range back to a few hundred thousand years and, in ideal conditions, back to nearly a million years; and uranium-series dating of calcareous tufas and travertines, with a possible age range of at least several hundred thousand years. Other dating methods that have been used in the Nile Basin include potassium–argon or argon–argon dating of certain crystals within volcanic rocks and tephra (ash) beds; palaeomagnetic dating of magnetic reversals in rocks or sediments that contain magnetic minerals; and amino acid racemisation dating of shell or wood samples.
Methods of relative rather than ‘absolute’ dating include the use of fossils, whether plants or animals, marine or continental, macroscopic or microscopic. On land pollen spectra have been widely used to reconstruct past changes in vegetation but are often rare or badly oxidised in arid environments (Lézine and Casanova, Reference Lézine and Casanova1989; Lézine et al., Reference Lézine, Casanova and Hillaire-Marcel1990, Reference Lézine, Hély, Grenier, Braconnot and Krinner2011). Pollen preserved in marine sediments also offer useful records of changes in the vegetation on land (Leroy and Dupont, Reference Leroy and Dupont1994, Reference Leroy and Dupont1997). Fossil vertebrate remains that have been independently dated in other localities can provide a useful chronology but often only at a coarse resolution, which is still better than guessing (Vrba et al., Reference Vrba, Denton, Partridge and Burckle1995). Marine sediment cores are usually dated using changes in the presence or absence of certain microfossil species together with analysis of changes in the ratio of the stable isotopes of oxygen, often supplemented by carbon isotope analysis. Terrestrial pollen grains are also found in marine sediments such as in the Nile Cone. Quaternary marine sediments across the world’s oceans now provide a relatively fine resolution record of variations in global ice volume, deep and shallow ocean water temperature and more local changes in sea water salinity (Lisiecki and Raymo, Reference Lisiecki and Raymo2005; Lisiecki and Raymo, Reference Lisiecki and Raymo2007; Railsback et al., Reference Railsback, Gibbard, Head, Voarintsoa and Toucanne2015), enabling individual marine isotope stages (MIS) to be placed in a numerical age sequence and related to past glacial–interglacial cycles and associated shorter climatic cycles. So, for example, MIS 1 extends from present to 14 ka, and MIS 2 from 14 ka to 29 ka (Table 4.2). The even numbers refer to the colder glacial or stadial climatic intervals and the uneven numbers to the warmer interglacial or interstadial climatic intervals (Gibbard and Cohen, Reference Gibbard and Cohen2008; Railsback et al., Reference Railsback, Gibbard, Head, Voarintsoa and Toucanne2015).
Table 4.2 Marine Isotope Stages (MIS) 1 to 20 (0–790 ka)
MIS 1: 0–14 | MIS 6: 130–191 | MIS 11: 374–424 | MIS 16: 621–659 |
MIS 2: 14–29 | MIS 7: 191–243 | MIS 12: 424–478 | MIS 17: 659–676 |
MIS 3: 29–57 | MIS 8: 243–300 | MIS 13: 478–533 | MIS 18: 676–712 |
MIS 4: 57–71 | MIS 9: 300–337 | MIS 14: 533–563 | MIS 19: 712–761 |
MIS 5: 71–130 | MIS 10: 337–374 | MIS 15: 563–621 | MIS 20: 761–790 |
Even MIS numbers are cold phases and uneven MIS numbers are warm phases.
Compiled from data in Williams et al. (Reference Williams, Dunkerley, De Deckker, Kershaw and Chappell1998), Walker (Reference Walker2005), Lisiecki and Raymo (Reference Lisiecki and Raymo2005, Reference Lisiecki and Raymo2007) and Gibbard and Cohen (Reference Gibbard and Cohen2008).
4.2 Geology
4.2.1 The Precambrian Legacy
We use the term Precambrian somewhat loosely to refer to rocks that are older than 540 Ma, after which complex living organisms became more widespread. The age of the Earth dates back to 4.6 billion (4.6 × 109) years ago, but we will confine our attention here only to rocks in and around what is now the Nile Basin and which date back to the last billion years or so, for which the evidence is modestly well dated and reasonably intensively studied (Vail, Reference Vail1976, Reference Vail1983, Reference Vail1985; Asrat et al., Reference Asrat, Barbey and Gleizer2001; Collins and Pisarevsky, Reference Collins and Pisarevsky2005; Fritz et al., Reference Fritz, Abdelsalam and Ali2013), as is that of the sediments derived from these rocks (Padoan et al., Reference Padoan, Garzanti, Harlavan and Villa2011; Garzanti et al., Reference Garzanti, Andò, Padoan, Vezzoli and El Kammar2015; Fielding et al., Reference Fielding, Najman and Millar2016, Reference Fielding, Najman and Butterworth2018). Early geologists working in Uganda, Ethiopia, Sudan and Egypt mapped and described a series of often highly eroded igneous and metamorphic rocks overlain with marked unconformity by younger sedimentary rocks (Andrew, Reference Andrew and Tothill1948; Whiteman, Reference Whiteman1971; Vail, Reference Vail1974, Reference Vail1978; see also the recent review by F. M. Williams, Reference Williams2016). Because these ancient rocks formed a foundation or basement beneath the younger relatively horizontal sedimentary formations, they became widely known as the basement rocks or the Basement Complex. Early workers in the 1960s inferred that the Basement Complex rocks had last been folded, faulted and metamorphosed during a major phase of mountain building termed the Pan-African Orogeny of 550–500 Ma, during the assembly of Gondwana, a vast supercontinent whose amalgamation was completed by 420 Ma. Gondwana (Fig. 4.1) was made up of a series of smaller coalescent landmasses, which later split off at different times from the parent supercontinent to become Africa, South America, India, Australia and Antarctica (Fig. 4.2).
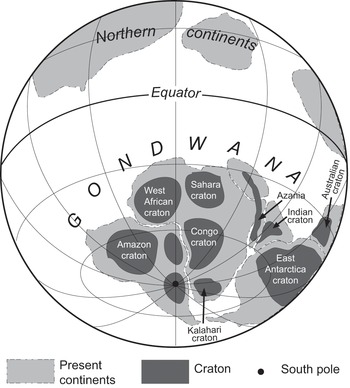
Figure 4.1 Gondwana at about 420 Ma ago. (Adapted from F. M. Williams, Reference Williams2016, Fig. 6.1.)
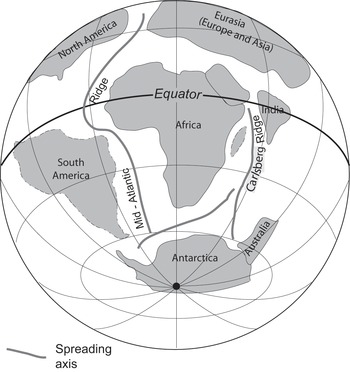
Figure 4.2 Gondwana at about 60 Ma ago. (Adapted from F. M. Williams, Reference Williams2016, Fig. 7.1.)
Once the Basement Complex rocks had been more rigorously dated and more thoroughly analysed and mapped, it became clear that the concept of a Pan-African Orogeny was oversimplified and no longer useful. We now refer to the East African Orogen (EAO) for the zone of former mountain building that extended from Arabia and Egypt in the north to Mozambique and Madagascar in the south. The style and tempo of mountain building and metamorphism was quite different in different parts of the EAO, with accretion of former slivers of both continental crust and ocean crust (Fritz et al., Reference Fritz, Abdelsalam and Ali2013). For the sake of simplicity and convenience, geologists distinguish what is loosely termed the Arabian–Nubian Shield in the north and the Mozambique Belt (MB) in the south. The southern sector of the Nile Basin includes small but significant outcrops of MB rocks, and the northern two-thirds of the Nile Basin are underlain by the so-called Nubian Shield rocks (Fig. 4.3). The East African Orogeny lasted from about 850 Ma to about 550 Ma (Fritz et al., Reference Fritz, Abdelsalam and Ali2013) and consisted of a number of orogenic episodes and associated metamorphism, separated by several phases of erosion and intrusion of coarse-grained igneous rocks such as granite, which were in turn subject to intense heat and pressure and metamorphosed to gneisses and other rocks, depending on their original composition.
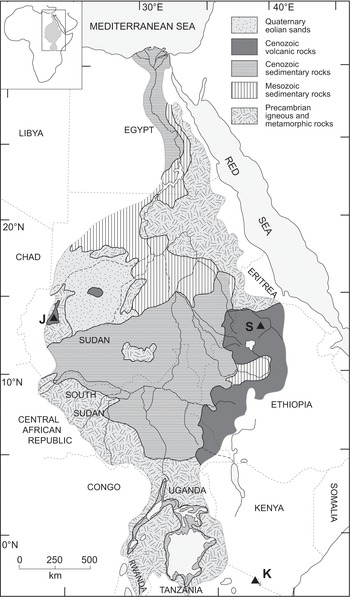
Figure 4.3 Geology of the Nile Basin. (Compiled by the author with data from Russell, Reference Russell1962: 1: 4,000,000 Geology Map; The Atlas of Africa, 1973: Geology Map, p. 29; (Vail, Reference Vail1978: 1:2,000,000 Geological Maps, North Sheet and South Sheet; Bureau de Recherches Géologiques et Minières, 1981: 1: 2,000,000 Geological Map of the Sudan; Vail, Reference Vail1985; Robertson Research and Geological Research Authority of the Sudan, 1988; Geological Research Authority of the Sudan, 1995; Schlüter, Reference Schlüter1997; Geological Research Authority of the Sudan, 2004: 1:2,000,000 Geological Map of the Sudan; Fritz et al., Reference Fritz, Abdelsalam and Ali2013; and F. M. Williams, Reference Williams2016: Fig. 4.2.)
Padoan et al. (Reference Padoan, Garzanti, Harlavan and Villa2011) used variations in the strontium and neodymium isotopic composition of sediments in the Nile Basin to identify the bedrock sources of the alluvium. Fielding et al. (Reference Fielding, Najman and Millar2016, Reference Fielding, Najman and Butterworth2018) expanded on this work by identifying the uranium–lead and hafnium isotope ages of zircons within Nile sediments throughout the Basin. In addition to defining in some detail the present-day sources of Nile alluvium, their work has also helped to refine our understanding of the different stages of development of the Arabian Nubian Shield (840–780 and 700–600 Ma), and the timing of the Gondwana supercontinent assembly (650–500 Ma).
The rocks formed during the EAO are important for several reasons. They are host to many precious and semi-precious metals and minerals that have been sought after from prehistoric times onwards. In the more humid tropical regions of the southern Nile Basin the Basement Complex rocks have been weathered to form moderately fertile soils that support (or once supported) a luxuriant cover of forest and woodland. They have also played a major role in the geomorphic history of the Nile. Many faults, shear zones and other tectonic lineaments formed during the Precambrian to Cambrian East African Orogeny have been re-activated at intervals during the past 500 million years (Roden et al., Reference Roden, Abdelsalam, Atekwana, El-Qady and Tarabees2011). Buried rift valleys in South Sudan are now filled with 10–15 km of sediments eroded from the mountains of southern Ethiopia that arose during the East African Orogeny. Ancient lineaments such as the Aswa wrench fault (see Chapter 13, Fig. 13.1) control the orientation of drainage in South Sudan. In northern Sudan and Egypt, the linear pattern of many Nile reaches is a direct reflection of the influence that these ancient structural controls exercise on Nile drainage patterns today, a topic we discussed in Chapter 2.
4.2.2 Northward Movement of the African Lithospheric Plate
It is convenient to begin this account with the break-up of Gondwana, which led to the separation of India–Australia–Antarctica from Africa some 180 Ma ago, followed by the splitting away of South America from Africa about 130 Ma ago. Africa then began to drift slowly northwards towards Eurasia, following a clockwise trajectory during the past 75 Ma (Habicht, Reference Habicht1979; Smith et al., Reference Smith, Hurley and Briden1981). In northern Nigeria and the Aïr Mountains of Niger there is a series of mid-Palaeozoic igneous intrusions and ring complexes that are older in the north and younger in the south, suggesting that they may have formed over one or more hotspots as the African plate drifted slowly northwards (Bowden et al., Reference Bowden, Van Breemen, Hutchison and Turner1976).
Another series of ‘Younger Granite’ igneous intrusions and ring complexes are aligned diagonally from SW to NE from the Cameroun Mountains of West Africa across the southern Sahara through southern Libya, northern Sudan and northern Eritrea to the Red Sea Hills. Ring complexes consist of a central granite core flanked by a series of concentric igneous rocks ranging from dolerites to gabbros. Differential erosion of the less resistant rocks means that concentric ridges of resistant rocks rise above annular- or crescent-shaped valleys flanked by alluvial fans and ephemeral stream channels and sometimes host now dry lakes. Such localities were attractive to prehistoric humans, as shown by the very long record of prehistoric human occupation at Adrar Bous ring complex in the Ténéré Desert of Niger (see Chapter 3, Fig. 3.6), extending from Early Stone Age to proto-historic times (Clark et al., Reference Clark and Gifford-Gonzalez2008a, Reference Clark, Brown, Dietler and Gifford-Gonzalez2008b, Reference Clark, Carter, Gifford-Gonzalez, Smith and Gifford-Gonzalez2008c; Gifford-Gonzalez, Reference Gifford-Gonzalez2008). The magnificent galleries of polychrome paintings of humans and herds of domestic cattle at Jebel ‘Uweinat ring complex, which straddles the border between Sudan, Egypt and Libya, are striking evidence of how important these mountains were to Neolithic desert pastoralists. Jebel ‘Uweinat also has a reliable supply of excellent fresh water in the form of several underground springs, and so has served as an occasional refuge for small bands of indigenous Tibu nomads early last century. Jebel Arkenu is a smaller ring complex 40 km northwest of ‘Uweinat, and was also visited by Neolithic and later herders who left behind some vivid rock paintings (Williams and Hall, Reference Williams and Hall1965). It is possible that some of the ring complexes in northern Sudan could also have been places of prehistoric refuge from the surrounding desert during times of somewhat wetter climate.
The western sector of North Africa moved into the dry tropical latitudes of the Northern Hemisphere during the late Mesozoic and early Cenozoic, resulting in widespread formation of evaporite deposits in Tunisia and Algeria (Coque, Reference Coque1962; Conrad, Reference Conrad1969), to be followed by Egypt and northern Sudan during Miocene times onwards. The inception of aridity in the Sahara stems from these times, and was interspersed by prolonged intervals when the prevailing climate was less arid, allowing Mediterranean plants to migrate south into the central and southern Sahara along long defunct waterways, and plants from the wet tropics to migrate northwards, where they now form relict populations in the mountain refugia of the Hoggar, Tibesti, Jebel Marra and the Aïr (see Chapter 3, Fig. 3.6) (Quézel and Martinez, Reference Quézel and Martinez1958–59, Reference Quézel, Martinez and Hugot1962; Quézel, Reference Quézel and Hugot1962; Beucher, Reference Beucher1971; Wickens, Reference Wickens1976a, Reference Wickens1976b; Ozenda, Reference Ozenda1977; Maley, Reference Maley, Williams and Faure1980, Reference Maley, Renault-Miskovsky and Semah2004, Reference Maley2010).
4.2.3 Palaeozoic and Mesozoic Sedimentation
During the long intervals following episodes of mountain building, such as from about 450 Ma onwards, much of North Africa and the region now occupied by the Nile Basin was subject to prolonged intervals of erosion and continental sedimentation, leading to widespread accumulation of sandstones such as the Mesozoic Nubian Sandstone Formation. These long intervals of erosion by former river systems were interspersed with occasional periods of intense cold and widespread glaciation, with the Cambro-Ordovician glaciations leaving a legacy of glacial sediments and glacially scoured rock pavements across Gondwana. The Ordovician glacial pavements in the Hoggar region of the central Sahara have only recently been exhumed from their sedimentary cover and are so well preserved that they resemble the most recent glacial landscapes of the late Quaternary (Beuf et al., Reference Beuf, Bijou-Duval and De Charpal1971). Not all parts of the region underwent erosion at the same time. On occasion the oceans flooded some of the areas at lower elevations or which were subject to sustained tectonic subsidence. Much of the Sahara and the Horn of Africa, especially Somalia and the Ogaden region of Ethiopia (F. M. Williams, Reference Williams2016) lay beneath the sea at intervals during the Cretaceous (Faure, Reference Faure1962a; Furon, Reference Furon1963). Marine Cretaceous sediments are found today at elevations approaching 2,000 m in the northern Aïr massif, indicating faulting and uplift after the seas receded (Faure, Reference Faure1959, Reference Faure1962b).
4.2.4 Cenozoic Tectonism, Volcanism and Sedimentation
The Cenozoic Era spans the last 65 million years of geological time. It marks the proliferation of land mammals following the demise of the dinosaurs at the end of the Mesozoic Era. As the African lithospheric plate moved north and began to impinge on Eurasia, portions of the crust became deformed and uplifted during the Hercynian Orogeny, which gave rise to the Atlas Mountains and the Alps. Portions of the previously stable Saharan shield also experienced uplift and associated volcanic activity, as in Jebel Marra in western Sudan and the Hoggar and Tibesti in the central Sahara. During the Palaeocene and Eocene great tracts of what is now the Sahara were forested and subject to a hot wet equatorial climatic regime. Miocene uplift and climatic desiccation led to erosion of the deep weathering mantle and exhumation of the irregular weathering front (Dresch, Reference Dresch1959; Greigert and Pougnet, Reference Greigert and Pougnet1967; Thorp, Reference Thorp1969; Williams, Reference Williams and Cloudsley-Thompson1984). The origin of the Sahara as a desert probably stems from about this time (Williams et al., Reference Williams, Abell, Sparks, Frostick and Reid1987; Williams, Reference Williams2014).
However, it would be misleading to think that the Miocene Sahara was as arid as it is today. During the late Miocene, a series of big rivers flowed from what is now the Chad Basin northwards, carving wide drainage channels in the southern Libyan Desert between Tibesti volcano to the west and three Nubian Sandstone plateaux located just to the east. Griffin (Reference Griffin1999, Reference Griffin2002, Reference Griffin2006, Reference Griffin2011) called these the Sahabi Rivers and inferred that during the time they flowed north across the present Libyan Desert to the southern coast of the Mediterranean, the climate in their upper catchment was relatively humid. This conclusion is consistent with the geochemical, faunal and floral evidence reported by Moussa et al. (Reference Moussa2016) of a mosaic of wetlands, savanna grasslands and woodlands in the Chad Basin during the late Miocene.
The late Miocene Mediterranean was a salt desert during what has been called the Messinian Salinity Crisis (Hsü et al., Reference Hsü, Montadert and Bernoulli1977; Hsü, Reference Hsü1983) dated to between 5.96 Ma and 5.33 Ma (Cosentino et al., Reference Cosentino, Buchwald and Sampalmieri2013). Desiccation of the Mediterranean may have been a consequence of tectonic movements but seems more likely to have been caused by fluctuating sea levels associated with the waxing and waning of ice caps in West Antarctica (Williams et al., Reference Williams, Dunkerley, De Deckker, Kershaw and Chappell1998; Williams, Reference Williams2014). During times of lower glacial sea levels, the shallow sill at the western end of the Mediterranean (the Straits of Gibraltar) would act as a dam and prevent free flow of Atlantic water into the Mediterranean Basin, and corresponding outflow of Mediterranean waters to the Atlantic. Given present rates of evaporation across the Mediterranean, a few short centuries would suffice to dry out much of the sea. Successive inflow and desiccation resulted in the accumulation of halite and other evaporites on the floor of the basin, eventually leading to the precipitation of a layer of salt up to about 1 km thick on the floor of the Mediterranean Basin. Repeated drying out of the Mediterranean Sea implies repeated lowering of river base level with concomitant deep fluvial entrenchment and, in the case of the Nile, formation of the deep fluvial canyon described in Chapter 2. It is likely that other big rivers also cut deep canyons at this time.
Tectonic movements were far from synchronous across the Nile Basin and adjoining areas. In Ethiopia uplift and associated widespread volcanic activity during the last 30 Ma seems to be related to the presence of a hotspot or intensely hot mantle plume beneath what is now Arabia and Ethiopia. Uplift led to crustal fracturing. Faults developed progressively along what is now the Red Sea, perhaps as early as 25 Ma, with sea-floor spreading commencing some 5 Ma ago. The Ethiopian and Afar Rifts began to open 15–10 Ma ago, but did not acquire their present form until during the late Pliocene and early Pleistocene. Uplift of what became the Ethiopian Highlands (and headwaters of the Blue Nile and Atbara Rivers) was accompanied by episodic subsidence in the Afar Rift to the east and in the lowlands of South Sudan in the west. Both of these localities became significant depocentres or zones of sediment accumulation, with 10–15 km of oil-bearing sediments accumulating in South Sudan beneath what is now the White Nile valley. In the arid Afar Rift there are sporadic sedimentary remains of once large lakes, as well as the fossil-bearing Pliocene and Quaternary deposits with their well-known hominin fossils.
Volcanic activity has persisted until the present day and, together with sporadic earthquakes, remains as much of a potential hazard for the modern inhabitants of Ethiopia as it must have been for their prehistoric forebears. There are also scattered volcanic hills in the arid deserts of Nubia and the Butana. The columnar basalts often appear very fresh and angular – perhaps because of minimal chemical weathering in this arid environment, but perhaps also because the eruptions were quite recent. On the slopes and summit of one such basalt hill rising above the dissected Nubian Sandstone tablelands 50 km east of Kerma on the Nile right bank in northern Sudan there are scattered Late Stone Age/Mesolithic and younger stone flakes. The previously horizontal Nubian Sandstone beds through which the basaltic lavas were extruded have been tilted around the margins of the volcano to angles of 15–20°.
Sandford (1933) had earlier described explosion craters and the denuded stumps of volcanoes southwest and northeast of Jebel ‘Uweinat on the border between Sudan, Egypt and Libya, noting that ‘both types were encircled by steeply tilted, hardened, and locally fused Nubian sandstone’ (Sandford, 1933, p. 47). Despite their very fresh appearance he concluded that ‘these explosion craters seem to be far older than they at first appear’ (Sandford, 1933, p. 49) but offered no suggestion as to their age. He commented briefly on the basaltic lavas and ‘volcanoes’ in the Bayuda desert between Berber and Merowe, which likewise intrude through the Nubian Sandstone and so are younger than Upper Cretaceous age.
4.2.5 Quaternary Climatic Fluctuations and Long-Term Desiccation
The Quaternary Period began 2.58 Ma ago and continues to this day. It consists of two Epochs of unequal duration: the Pleistocene (2.58 Ma to 11.7 ka) and the Holocene (11.7 ka to present) (Cohen et al., Reference Cohen, Finney, Gibbard and Fan2013). There is much talk of defining a new Epoch – the Anthropocene – starting at a yet to be decided date, but most probably about the middle of last century (Waters et al., Reference Waters2016). For present purposes, we will consider that we are still in the Holocene.
The Quaternary is characterised by rapid fluctuations in global temperature and global ice volume, reflected in multiple glacial–interglacial cycles. These cycles were modulated by three astronomical cycles (Fig. 4.4), all of which control the amount of solar radiation received in the upper atmosphere, which in turn controls the temperature at the Earth’s surface (Williams et al., Reference Williams, Dunkerley, De Deckker, Kershaw and Chappell1998; Williams, Reference Williams2014). The relative influence of these three cycles on global climate has varied through time and may be discerned from the record of marine sediment cores. It is useful to discuss each in turn since they have all exerted an important influence upon the Nile Basin.

Figure 4.4 Orbital fluctuations. (After Williams, Reference Williams2014: Fig. 3.6.)
Earth follows an elliptical path around the sun each year. At present, when Earth is closest to the Sun (termed the perihelion), the distance between Earth and Sun is 147.1 million km. When farthest from the sun (termed the aphelion) the distance is 152.1 million km. The shape of this elliptical path changes over time, being sometimes more and sometimes less elliptical. This cyclical change is known as orbital eccentricity, lasts 96,600 years, and leads to a 3.5% variation in solar radiation received in the outer atmosphere.
The tilt of Earth’s axis – at present 23° 27′ – also varies, from a maximum tilt of 24° 36′ to a minimum tilt of 21° 59′. When the inclination is greatest, summers in higher latitudes tend to be hotter and winters colder. Times of minimum tilt are times of mild winters and mild summers. This obliquity cycle lasts 41,000 years and exerts a powerful control over seasonality.
The third cycle is the precessional cycle and over the last million years may have varied in length from 16.3 ka to 25.8 ka, but over the past 10 million years is generally considered to have varied between 23 ka and 19 ka. This cycle reflects the changing season of the year when Earth is closest to the Sun and is controlled by the direction in which the spin axis of Earth points in space.
In the late Pliocene, the dominant cycles evident in marine sediment cores are the 19 ka and 23 ka precessional cycles. The 41 ka obliquity cycle then became dominant until about 0.7 Ma, after which the 100 ka orbital eccentricity cycle became dominant (Lisiecki and Raymo, Reference Lisiecki and Raymo2005; Clark et al., Reference Clark, Archer, Pollard, Blum, Rial, Brovkin, Mix, Pisias and Roy2006; Lisiecki and Raymo, Reference Lisiecki and Raymo2007). In terms of direct impact on climate, the last 0.7 Ma were subject to high-amplitude but low-frequency fluctuations, while the preceding time was subject to lower amplitude but higher frequency fluctuations. In short, glacial–interglacial cycles lasted about 100 ka after about 0.7 Ma ago, but were shorter and less severe before then. During the last 0.7 Ma, each glacial cycle was characterised by a long, slow, saw-toothed build-up to extreme cold, maximum ice volume and minimum sea level, followed by a short, rapid warming and a brief interglacial interval of warmer and often wetter climate within the intertropical zone. Our present interglacial effectively began at the start of the Holocene 11,700 years ago, although there were brief intervals of warmer global climate in the preceding few thousand years before then.
The Quaternary was characterised by the accumulation of large ice caps over North America and NW Europe roughly 2.6 Ma ago, followed by the waxing and waning of these ice caps (Williams et al., Reference Williams, Dunkerley, De Deckker, Kershaw and Chappell1998). The repercussions included global sea level fluctuating in relation to how much water had been abstracted from the oceans to feed the growing ice caps, and how much glacial melt-water was released into the oceans once the ice melted. As one might expect, the glacial intervals were times when temperatures across the continents were generally colder than today, as were the sea surface temperatures. Lower sea surface temperatures were associated with reduced evaporation from the tropical oceans, leading to a reduction in precipitation over land, at least within the intertropical regions (Galloway, Reference Galloway1965a; Williams, Reference Williams2014). Tropical lakes dwindled in size, some becoming more saline or drying out altogether (Tillet, Reference Tillet1997; Gasse et al., Reference Gasse, Chalié, Vincens, Williams and Williamson2008; Williams, Reference Williams2014). Hitherto perennial rivers became more seasonal or even ephemeral. Reduced precipitation and lower glacial temperatures caused the tropical rain forests to shrink and become fragmented (Flenley, Reference Flenley1979; Anhuf et al., Reference Anhuf, Ledru and Behling2006), with corresponding habitat fragmentation and isolation of some of the less mobile mammals, birds and insects (Moreau, Reference Moreau, Howell and Bourlière1963). In the seasonally wet tropics once vegetated and stable dunes became bare and mobile, as along the southern margin of the Sahara (Grove, Reference Grove1958; Grove and Warren, Reference Grove and Warren1968; Talbot, Reference Talbot, Williams and Faure1980). With stronger and gustier winds and a reduced plant cover it was easier to mobilise and transport fine soil and sediment particles, and dust storms were also more frequent (McGee et al., Reference McGee, Broecker and Winckler2010).
With a return to warmer wetter conditions, there was a corresponding expansion of the tropical and montane rain forest and a stabilisation of previously active sand plains and dune fields. Lake levels rose once more and rivers became less seasonal. Plants and animals expanded their habitat into previously arid or semi-arid areas, and parts of the southern Sahara became covered in savanna woodland and grassland, most recently during the first half of the Holocene (Williams, Reference Williams and Gifford-Gonzalez2008). During the past 5,000 years conditions became arid once more. Many small lakes that were scattered across the Sahara dried out completely, as regional water tables sank well below the surface, and once perennial or seasonal streams and rivers ceased to flow (Hoelzmann et al., Reference Hoelzmann, Gasse, Dupont, Battarbee, Gasse and Stickley2004; Williams, Reference Williams2014). The former river channels in the eastern Sahara detected by side-scanning radar are good examples of such now defunct drainage systems (McCauley et al., Reference McCauley, Schaber and Breed1982, Reference McCauley, Schaber and McHugh1986; Breed et al., Reference Breed, McCauley, Davis, Frostick and Reid1987). The oldest of these may date back to Oligocene time while the youngest were still active during the Lower Palaeolithic/Early Stone Age, being associated with Acheulian hand axes and other bifacial tools (McCauley et al., Reference McCauley, Schaber and McHugh1986). The seasonal dust storms such as the haboob storms in the Nile Valley and the Harmattan dust storms in West Africa became more active, the latter attracting the attention of Dobson (Reference Dobson1781) and the young Charles Darwin (Reference Darwin1846) as well as many later workers (McTainsh, Reference McTainsh1980, Reference McTainsh1984, Reference McTainsh1987; McTainsh and Walker, Reference McTainsh and Walker1982; Washington et al., Reference Washington, Todd and Lizcano2006).
Active sand dunes and sand plains cover about one fifth of the Sahara today but during drier climatic phases dunes were active more than 500 km south of their present limits in West Africa and the Sudan (Fig. 4.5) (Grove and Warren, Reference Grove and Warren1968; Talbot, Reference Talbot, Williams and Faure1980; Swezey, Reference Swezey2001). Wind-blown sands are present in many of the Neogene well sections examined by Michel Servant in the Chad Basin (Servant, Reference Servant1973; Servant and Servant-Vildary, Reference Servant, Servant-Vildary, Williams and Faure1980). There is a close association between former rivers, sand dunes and closed sedimentary basins in North Africa (Williams, Reference Williams2015), and the same is true of the Nile Basin. Quaternary and older rivers flowing from the upland drainage divides between topographic depressions ferried gravel, sand and finer particles across the hill slopes and down to the valley floors. The finer material (silt and clay) provided the source material for desert dust, much of which was blown out to sea (Morales, Reference Morales1979; Schütz et al., Reference Schütz, Jaenicke, Pietrek and Péwé1981) or even as far afield as the Amazon Basin (Swap et al., Reference Swap, Garstang, Greco, Talbot and Kallberg1992; Prenni et al., Reference Prenni, Petters and Kreidenweis2009). The resistant quartz sand grains at the distal end of desert river channels were soon reworked by wind and in due course formed desert dunes of great symmetry and multiple forms (Cooke et al., Reference Cooke, Warren and Goudie1993; Warren, Reference Warren2013). There is always a type of geomorphic tug-of-war between wind and water in arid areas. Sometimes the rivers flow strongly and erode the dunes. At other times wind action gains the upper hand and former stream channels are buried beneath wind-blown sand. Given enough time, wind-blown sand will gradually obliterate former lakes and ephemeral stream channels, as in the case of the concealed river channels revealed by side-scanning radar to the west of the Egyptian Nile (Breed et al., Reference Breed, McCauley, Davis, Frostick and Reid1987).
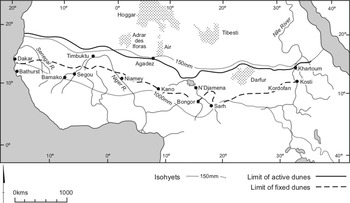
Figure 4.5 Map showing the limits of active and fixed dunes in and beyond the Sahara. The present-day limit of active dunes is bounded by the 150-mm isohyet. Fixed dunes extend up to 500 km south of the Sahara, locally into areas that now receive 1000 mm of mean annual rainfall. (Modified from Vail, Reference Vail1978; Grove, Reference Grove, Williams and Faure1980; Mainguet et al., Reference Mainguet, Canon, Chemin, Williams and Faure1980; and Talbot, Reference Talbot, Williams and Faure1980.)
Both the White Nile and the main Nile show signs of temporary channel blockage by wind-blown sand during drier intervals in the late Quaternary (Butzer and Hansen, Reference Butzer and Hansen1968; Williams et al., Reference Williams, Williams and Duller2010). Shifting wind belts during the late Quaternary fashioned linear dune systems, which today show slightly different orientations and degrees of soil development, as is clearly evident in the Qoz west of the lower White Nile in Kordofan Province (Warren, Reference Warren1970). (Qoz is a general Arabic term for sand dune or sand plain). Some of the linear dunes west of the Nile are many hundreds of km long, and the remarkable Qoz Abu Dulu dune extends N–S for more than 500 km and, in common with many dunes in this area, appears to be a polygenic dune (Williams et al., Reference Williams, Williams and Duller2010, Reference Williams, Duller and Williams2015a).
In contrast to North America and NW Europe, Quaternary glaciations were never a major factor in fashioning the landscapes of the Nile Basin. There were small ice caps and valley glaciers in the Ethiopian Highlands, notably in the Bale Mountains of southern Ethiopia and in the Semien Mountains close to the headwaters of the Blue Nile and Atbara Rivers. In Uganda, the glaciers that are still present on Mount Ruwenzori expanded and Mount Kivu (Livingstone, Reference Livingstone1975, Reference Livingstone, Williams and Faure1980) was also glaciated during what is called the Last Glacial Maximum (LGM) some 21,000 ± 2,000 years ago (Mix et al., Reference Mix, Bard and Schneider2001). In the Sinai Desert of Egypt at least one of the high mountains shows signs of having been glaciated in geologically recent times (Messerli and Winiger, Reference Messerli, Winiger, Rognon, Williams and Faure1980) and the high Atlas Mountains in northwest Africa had a more extensive cover of snow and ice during the LGM (Messerli, Reference Messerli1972; Messerli et al., Reference Messerli, Winiger, Rognon, Williams and Faure1980; Messerli and Winiger, Reference Messerli and Winiger1992). The Hoggar Mountains in the central Sahara also show signs of several phases of late Quaternary glacial erosion and deposition (Rognon, Reference Rognon1967). It seems unlikely that Jebel Marra near the western edge of the Nile Basin was ever covered in ice, but frost shattering during times when temperatures were up to 8–12°C lower than today may have been quite widespread across the more arid parts of the Nile Basin. Frost shattering was probably responsible for some of the highly angular blocky rubble seen by the author on the summit of a small basalt volcano located at 19°42.101′N and 30°40.379′E in the desert of northern Sudan. Frost shattering and downslope movement of angular rubble were certainly active in the Semien Mountains during the LGM, as discussed in Chapter 7.
4.3 Soils
Figure 4.6 shows the main types of soil found within the Nile Basin and Table 4.3 lists their main characteristics. A soil may be defined very simply as weathered rock or sediment that has been altered from its original state as a result of biological processes resulting from plant, animal and microbial activity. Soils can be discontinuous or can form more or less continuous mantles across the landscape. Soil scientists have traditionally identified five main factors that are responsible for soil formation: parent material, topography, biological activity, climate and time (Jenny, Reference Jenny1941; Kubiëna, Reference Kubiëna1950; United States Department of Agriculture, 1951; Mohr and Van Baren, Reference Mohr and van Baren1959; Mohr et al., Reference Mohr, van Baren and van Schuylenborgh1972; Duchaufour, Reference Duchaufour1978; Paton, Reference Paton1978; Paton et al., Reference Paton, Humphreys and Mitchell1995; Birkeland, Reference Birkeland1999; Retallack, Reference Retallack2001; Williams, Reference Williams2014). Parent material and topography have the greatest influence on soil characteristics during the early stages of soil development. Paton (Reference Paton1978, p. 96) termed these two factors the ‘passive factors’ of soil formation. As time progresses, climate and biological activity (Paton’s ‘active factors’) exert an increasingly important influence on soil development. Climate refers to soil climate, which is ultimately controlled by the local climatic regime, and includes both the soil moisture regime and the soil temperature and evaporation regimes. Soil moisture is controlled by soil permeability and by the soil infiltration capacity. Different rock types, whether igneous, metamorphic or sedimentary rocks, exert quite different influences on the physical and chemical characteristics of immature soils. As a result, it is possible to distinguish quite easily between young soils developed on the most common types of rock such as granite, basalt, sandstone, siltstone and limestone, discussed in Section 4.3.1.

Figure 4.6 Soils of the Nile Basin. (Compiled by the author with data from Greene, Reference Greene and Tothill1948; Russell, Reference Russell1962: 1: 4,000,000 Soils Map; Mitchell, Reference Mitchell and Cloudsley-Thompson1984, Fig. 4.3; Buursink, 1971; Blockhuis, Reference Blokhuis1993; Nile Basin Initiative, 2012; and author’s unpublished observations.)
Table 4.3 Major soil groups in the Nile Basin
Soil group | Characteristics |
---|---|
Andosols | Soils formed in volcanic ash, with abundant volcanic glass (Andisols) |
Arenosols | Sandy soils with minimal texture contrast and weak or no soil horizons (Entisols) |
Ferralsols | Soils rich in iron or aluminium (Ultisols) |
Fluvisols | Young soils in alluvial deposits still showing signs of alluvial stratification (Entisols) |
Lithosols | Shallow soils, composed mainly of unweathered rock fragments |
Regosols | Soils formed on deep unconsolidated recently deposited sands or alluvium (Entisols) |
Vertisols | Heavy dark churning clay soils with deep vertical cracking in the dry season. Contain abundant swelling clay minerals (notably, montmorillonite). Variable salinity and alkalinity (Vertisols) |
Based on author’s observations with names adapted from World Reference Base for Soil Resources (2010).
A long-acknowledged and distinctive feature of all soils worldwide is that they show some degree of vertical differentiation and are arranged in roughly horizontal or gently sloping soil layers traditionally known as soil horizons (Joffe, Reference Joffe1949; Kubiëna, Reference Kubiëna1950; United States Department of Agriculture, 1951; Mohr and van Baren, Reference Mohr and van Baren1959; Soil Survey Staff, 1960; Mohr et al., Reference Mohr, van Baren and van Schuylenborgh1972; Buol et al., Reference Buol, Hole and McCracken1973; Duchaufour, Reference Duchaufour1978; Paton et al., Reference Paton, Humphreys and Mitchell1995; Birkeland, Reference Birkeland1999; Retallack, Reference Retallack2001; Soil Survey Staff, 2010; Williams, Reference Williams2014). The near-surface soil layers tend to be darker in colour because more enriched in organic matter derived from the plants growing in the soil. This topsoil is termed the ‘A horizon’ and will become thicker and darker over time as the plant cover becomes well established. Beneath the ‘A horizon’ is a layer of soil usually with a higher clay content than the topsoil and usually with brighter colours if the site is well drained; this layer is called the ‘B horizon’. Beneath the ‘B horizon’ is the parent sediment or ‘C horizon’, which is generally altered from its initial state but still retains signs of its depositional origin. If the soil is developed directly over bedrock, the ‘B horizon’ will overlie what is termed the ‘R horizon’.
The role of the soil fauna has long been recognised. Darwin (Reference Darwin1881) was the first to quantify in a systematic fashion the soil-moving abilities of earthworms. Seven years later, Drummond (Reference Drummond1888) argued very persuasively that in terms of their soil moving capacity, termites were the tropical analogues of earthworms in more temperate climatic regions. Many fossil soils in arid North Africa show evidence of former termite activity (Conrad, Reference Conrad1959), with implications for soil classification. Not all soils in tropical areas fall neatly into the ABC system of soil horizons recognised by soil scientists. For instance, soil profiles developed over weathered granite in tropical Africa and tropical northern Australia are a result of the activities of the soil fauna – in this case termites – and have been described as having M, S and W horizons (Nye, Reference Nye1954, Reference Nye1955; Watson, Reference Watson1961, Reference Watson1962, Reference Watson1964; Williams, Reference Williams1968a, Reference Williams, Davies and Williams1978). Many other soils also reflect the influence of the soil fauna, especially from ants, earthworms and burrowing mammals (Paton, Reference Paton1978; Johnson, Reference Johnson1993; Paton et al., Reference Paton, Humphreys and Mitchell1995). The M horizon is a migratory mineral layer of mainly quartz coarse sand and very fine quartz granules up to 3 mm in size, and often up to 50 cm thick. The M horizon overlies the S horizon or stone layer, which may be enriched towards the base in clay-sized particles. The stones consist of mostly quartz fragments over about 3 mm in size derived from quartz veins within the weathered granite. The W horizon refers to the granite weathering profile which grades down into fresh rock (Fig. 4.7). Such soils have formed as a result of the mining activities of mound-building worker termites, which travel down their subterranean galleries until they reach the moist weathering front where they forage for silt-, clay- and sand-sized particles with which to construct their mounds during the wet season. The sand grains act as bricks and the wet mud as mortar. Once abandoned, the mounds are eroded by rainstorms and soon break down into their constituent particles. The finer particles are rapidly washed downslope by rain splash (Williams, Reference Williams1969) and overland flow, leaving the sands and fine quartz granules behind to form the M horizon. The long-term effect of the termite mining activities at the weathering front is to leave a concentrated subsurface residue of any quartz particles too big for the worker termites to carry, usually about 2–3 mm. This residual layer comprises the S horizon or stone layer and may contain mineral particles such as gold.
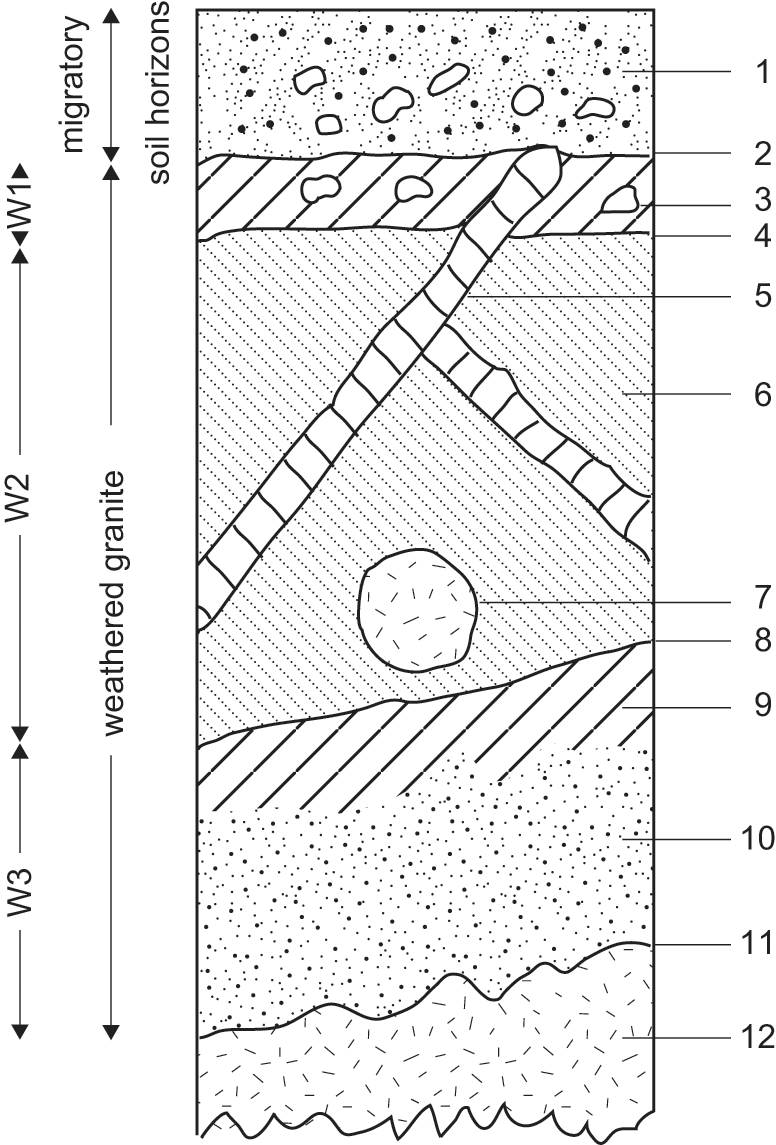
Figure 4.7 Schematic profile of soil developed on weathered granite. (Based on the author’s unpublished observations.) 1. Sand over quartz stone layer. 2. Gradual irregular boundary. 3. Stony red clay loam. 4. Diffuse wavy boundary. 5. Undisturbed quartz or pegmatite veins within weathered granite. 6. Reddish-brown or yellowish-red sandy clay loam. 7. Whitish-grey granite remnant. 8. Clear smooth boundary. 9. Clay-enriched (illuviated) surface of W3 zone. 10. White or grey coarse sandy loam. 11. Diffuse irregular boundary. Fresh granite.
4.3.1 Soils Formed on Weathered Bedrock and Associated Colluvium
Roughly half of the soils found within the Nile Basin have been formed in situ on weathered bedrock and are termed sedentary soils. Colluvial sediments derived from the adjacent weathered bedrock are often found next to areas of sedentary soil and may support skeletal or immature soils. Colluvial materials can range from very coarse-grained to fine-grained, and consist of sediments that have moved downslope under the influence of gravity, either rapidly as with debris-flow and rock-fall deposits or slowly as with fine sediments subject to soil creep and other forms of slow mass movement.
As noted earlier, the physical and chemical properties of recently formed sedentary soils closely reflect the nature of the parent rock from which they are derived. For instance, soils developed on weathered granites are often slightly acidic and usually consist of a topsoil of quartz sand over a more clay-rich sandy subsoil over weathered rock in which some or all of the original rock fabric is still visible. In those seasonally wet tropical areas where mound-building termites are active, the granite-derived soils can consist of a three-layered soil profile, with a topsoil of coarse quartz sand over a stone layer of angular quartz over the weathered parent rock (Fig. 4.7). Such soils are not particularly fertile and will tend to support a cover of sparse trees and tall grasses. Around the periphery of many granite hills in the Sudan there is often an aureole of sandy clay, which retains moisture during the dry season and supports a much denser band of vegetation (Ruxton, Reference Ruxton1958). The reason for this is that mechanical eluviation by subsurface lateral flow (Kirkby and Chorley, Reference Kirkby and Chorley1967) moves the finer clay and silt particles down slope and through the sandy topsoil until they reach the bottom of the slope, at which point the hydraulic gradient diminishes rapidly and the fine particles are deposited within the voids around the coarser sand grains. In the hot wet tropical regions in the south of the Nile Basin, the granites are usually deeply weathered, and have often undergone one or more later phases of vertical incision and valley widening, resulting in the exhumation of the irregular former weathering front (Fig. 4.8). The landscapes formed in this way consist of low granite tors and tall granite hills or inselbergs, rising above gently sloping plains and wide alluvial flats (Fig. 4.9).
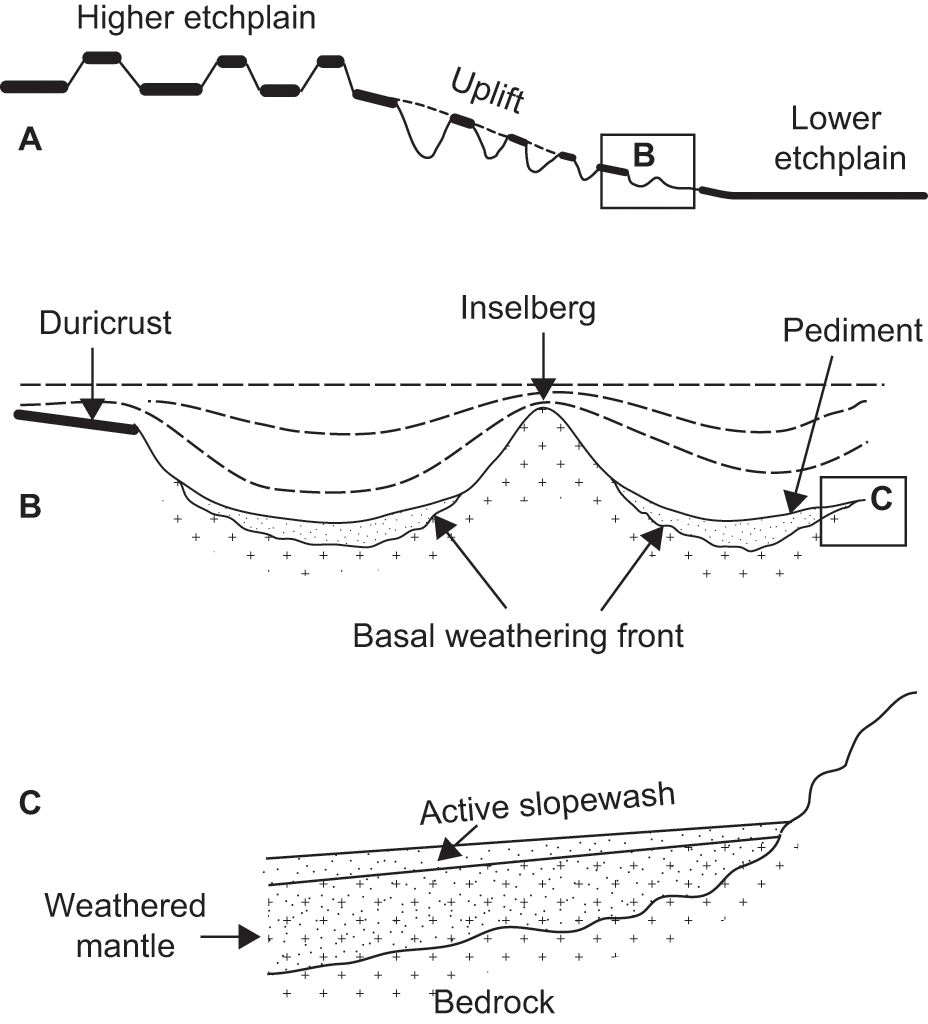
Figure 4.8 Deep weathering of granite rocks and exhumation of the weathering front, southern Nile Basin. The exhumed deep weathering front is termed an etchplain. The duricrust is an iron-enriched caprock. An inselberg is a hilly erosional remnant. A pediment is a gently sloping rock-cut surface at the base of a plateau or an inselberg. The basal weathering front is the transitional zone between fresh and deeply weathered bedrock (weathered mantle). (Based on the author’s unpublished observations.)
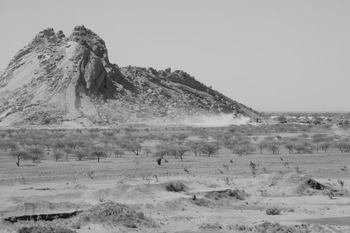
Figure 4.9 Granite inselberg, central Sudan.
As one might expect, soils formed recently on quartz sandstone consist of a fairly uniform layer of quartz sand that lies directly over the parent sandstone. In the drier parts of the Nile Basin, these sandy soils may also contain lenses of gravel deposited by local ephemeral stream channels. In Nubia and southern Egypt, the Nubian Sandstone covers a huge area and is a major regional aquifer, so that some modest cultivation is possible despite the lack of plant nutrients in these sandy soils. What nutrients there are have often come from wind-blown dust, which frequently consists of calcareous clay particles that are subsequently washed into the sandy soils during occasional rainstorms or during wetter intervals in the recent past. In the far south of the Nile Basin in localities where the climate is perennially wet or where there is a prolonged wet season, the sandstone is often highly weathered and the soils formed above it contain abundant concretions of iron-cemented sand. These red ironstone soils have a variety of local names and are often termed laterites or ferralsols. [The term laterite has been used to describe such a huge variety of soil materials of quite different origins (Paton and Williams, Reference Paton and Williams1972) that the discerning reader had best declare a closed season on the use of this term.]
Certain fossil soils developed over Nubian Sandstone in arid northern Sudan have the vesicular honeycomb structure characteristic of soils once inhabited by termites. In order to survive, termites need to forage for grass or wood, depending on the species, and in order to construct their galleries need access to moist clay or silty clay, all of which presuppose a climate somewhat less arid than at present in what is today known as Nubia (Williams, Reference Williams2012b). Efforts to obtain optically stimulated luminescence (OSL) ages for these soils have proven difficult to interpret, yielding ages ranging between late Pleistocene and middle Holocene (ca. 52–6.5 ka), which may simply indicate that termites were last active in this now arid environment early in the Holocene. We discuss this further in Chapter 14.
Beds of siltstone, shale or mudstone are sometimes sandwiched between more massive beds of sandstone, and being easier to erode than sandstone often form relatively flat depressions or valleys, depending on the thickness of the siltstone beds. The soils developed on siltstone are often quite variable and may be overlain by a transported layer of colluvial sandstone, but are in all cases fine-grained and usually richer in plant nutrients than the sandstone soils. In parts of Nubia local villagers quarry the buff and purple siltstones and use them to coat the walls of their mud-brick houses. Some of the siltstone soils contain gypsum, and modest quantities of gypsum can improve the permeability of heavy clay soils.
In the drier northern regions of the Nile Basin and in places along the main Nile valley there are localised outcrops of limestone. The soils developed on limestone vary considerably, depending on whether the parent rocks consist of muddy limestone, which weathers to calcareous clay, or very pure limestones, which give rise to thin and highly calcareous soils unless there is a significant contribution from wind-blown dust. Provided that there is a protective capping of desert pavement, the dust layer beneath the surface lag gravel can become thicker over time and can eventually form well-developed soils. (A desert pavement is a more or less continuous thin surface layer of stones that protects the underlying soil or sediment from erosion.)
By far the most fertile soils in the Nile Basin are those derived from volcanic rocks. The Ethiopian headwaters of the Blue Nile and the Atbara rise amidst the basaltic lava flows of the Ethiopian Highlands, as do the headwaters of the Sobat, which carries a suspension load of mud from Ethiopia to the White Nile, and the headwaters of the Rahad and Dinder Rivers, which today flow into the lower Blue Nile but may once have flowed directly across the alluvial plains of central Sudan to join the White Nile directly. We discuss these alluvial soils in Section 4.3.2; our concern here is with soils formed directly from basalt. In well-drained sites the basaltic soils are well aerated and the iron content sufficiently well oxidised to give rise to vivid red clay soils with the dominant clay minerals kaolinite and illite. In less well drained sites the dominant clay mineral is smectite (montmorillonite) and the soils are dark grey or dark grey brown cracking clays or vertisols, which expand when they are wet and shrink and crack as they dry out. These soils are widespread in the Ethiopian Highlands and are easily eroded during the intense rainstorms of the summer monsoon, bringing silt and clay to the lowlands of central Sudan and the flood plains of the Blue Nile, Atbara and main Nile, to which we now turn.
4.3.2 Alluvial Soils
At intervals throughout the Quaternary, and most recently during the last 15,000 years of the terminal Pleistocene and Holocene (Talbot et al., Reference Talbot, Williams and Adamson2000), the Ethiopian tributaries of the Nile have ferried a suspension load of silt and clay to the main Nile during the course of the Ethiopian summer monsoon season. The relatively narrow flood plain of the Nile has been built up and periodically eroded throughout this time, leaving a series of alluvial terraces as witness to these alternating episodes of alluvial sedimentation or aggradation, and fluvial incision or degradation (Hull, Reference Hull1896; Butzer and Hansen, Reference Butzer and Hansen1968; Butzer, Reference Butzer, Williams and Faure1980). The soils that have subsequently developed on these alluvial silts and clays (Table 4.3) reflect their combined volcanic and alluvial inheritance, and today consist of the dark cracking clays or vertisols (Fig. 4.10) that are such a characteristic feature of the arable lands of the Egyptian Nile Valley (Butzer and Hansen, Reference Butzer and Hansen1968; Said, Reference Said1981, Reference Said1993; Embabi, Reference Embabi2004) and of the Gezira plain between the Blue and White Nile rivers in central Sudan (Tothill, Reference Tothill1946a, Reference Tothill and Tothill1948; Sir Alexander Gibb & Partners, Reference Gibb1954; Jewitt, Reference Jewitt1955; Beinroth, Reference Beinroth1966; Drover, Reference Drover1966; De Vos and Virgo, Reference De Vos and Virgo1969, Buursink, Reference Buursink1971; Fadl, Reference Fadl1971; Gunn, Reference Gunn, Williams and Adamson1982; Williams et al., Reference Williams, Adamson, Abdulla, Williams and Adamson1982; Blockhuis, Reference Blokhuis1993; Williams et al., Reference Williams, Duller and Williams2015a). These soils are often self-mulching in that they develop a granular surface layer (Fig. 4.10), allowing them to retain moisture during the dry season. They generally have a moderate to high content of clay-sized particles (< 2 μ) and a correspondingly high cation exchange capacity. Although mostly non-saline and non-alkaline, in some sites such as shallow depressions or former fluvial embayments or may’a that were once flooded but have since dried out, the alluvial clays can be both saline and alkaline and may have a massive surface crust. Such soils occur sporadically along the lower White Nile valley, and reflect the flood history of that river, with its very gentle flood gradient of 1:100,000 (Williams, Reference Williams1968b).
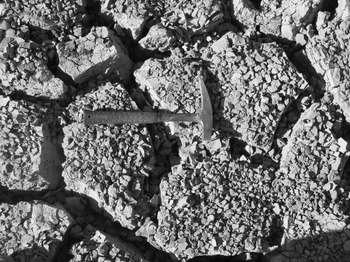
Figure 4.10 Cracking clay soil, Blue Nile Valley, Sudan.
One still unresolved question is the origin of the clays at higher elevations between the Blue and White Nile Rivers (Sir Alexander Gibb & Partners, Reference Gibb1954; Gunn, Reference Gunn, Williams and Adamson1982; Williams et al., Reference Williams, Adamson, Abdulla, Williams and Adamson1982), as well as the widespread clay plains situated east of the Blue Nile (Bunting and Lea, Reference Bunting and Lea1962; Ruxton and Berry, Reference Ruxton and Berry1978). Are these clays ancient alluvial clays deposited by the Blue Nile when it once flowed at much higher elevations on leaving the Ethiopian Highlands? Or are they clays formed in situ by weathering of the underlying bedrock and later moved downslope by variable amounts through processes of slow mass movement? Certainly, there is good field evidence that some of these clays do indeed appear to be part of a deep weathering profile grading down into the underlying bedrock (Hunting Technical Services, 1964), but we cannot rule out an alluvial origin for at least some of the higher-level clays located west of the present Blue Nile between the latitudes of Roseires (11.8°N, 34.4°E) and Sennar (13.4°N, 33.2°E) and extending out to the White Nile Valley above an elevation of 386 m.
4.3.3 Dune Soils
Soils that are developed on sand dunes initially have few obvious soil horizons and support meagre plant cover. However, as time progresses, the previously active dunes become stabilised by vegetation and trap calcareous desert dust particles which are then washed into the porous sand to form a clay-rich substrate. This process can be quite rapid: a matter of decades rather than centuries, depending on the dust flux (Williams, Reference Williams2014). Analysis of the strontium and neodymium content of such soils shows that dust storms are the main contributors of the clay and calcium carbonate (Woodward et al., Reference Woodward, Macklin and Fielding2015a). Close inspection of many vegetated dunes shows multiple clay bands running parallel to the original bedding. Once these bands coalesce the outcome is a topsoil of quartz sand over the clay-rich subsoil in which calcium carbonate concretions are often very abundant. Such concretions are at first soft and quite diffuse, but over time become hard and may form cylindrical coatings (‘rhizocretions’) around tree roots (Williams, Reference Williams1968c; Amit et al., Reference Amit, Lekach, Ayalon and Porat2007; Williams, Reference Williams2014; Williams et al., Reference Williams, Duller and Williams2015a). At a later stage the individual concretions may become cemented into calcrete, a very hard, massive and impermeable layer of calcium carbonate (Williams, Reference Williams2014). Calcretes are found near the base of some of the dunes east of the lower White Nile at depths of 1.5–2.0 m and are associated with former ponds between dunes (Fig. 4.11) or the now buried margins of former White Nile lakes (Williams, Reference Williams1968c; Williams et al., Reference Williams, Adamson, Abdulla, Williams and Adamson1982) discussed in Chapters 8 and 11.
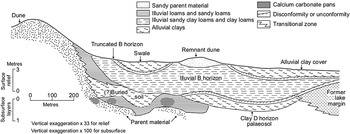
Figure 4.11 Dune soil catena east of the lower White Nile, central Sudan. (Modified from Williams, Reference Williams1968c), showing polygenetic and truncated soil profiles.)
4.3.4 Desert Soils, Polygenic Soils and Fossil Soils
Desert soils in the Nile Basin tend to be shallow, with limited horizon development, and are often both saline and alkaline. They are easily eroded unless protected by a surface layer of gravel (‘desert pavement’) or a hard surface crust. In some instances, the desert pavement may overlie one or more resistant bands of finer sediment formed during earlier intense and very localised desert rainstorms. These sediment layers are often finely laminated, reflecting deposition from overland flow. In addition, a thin layer of fine silt and clay particles may form an impermeable crust, promoting further runoff; such crusts are usually short lived. These sediment layers may also become cemented with silica, iron or calcium carbonate to form ‘hardpans’.
Soils developed on stable land surfaces in arid areas may over time develop distinct soil profiles indicative of past fluctuations in precipitation (Amit et al., Reference Amit, Lekach, Ayalon and Porat2007, Reference Amit, Enzel, Grodek, Crouvi, Porat and Ayalon2010). The depth to gypsum within the soil may be a guide to former precipitation (Retallack and Huang, Reference Retallack and Huang2010). Both the amount and depth of soil carbonate within a given dryland soil may also be indicative in a very general sense of the climatic conditions under which it formed (Williams, Reference Williams2014, pp. 276–278). The problem here is a variant on the well-known geomorphic conundrum of magnitude versus frequency. Is it the events of low frequency but high magnitude that do most of the work? Or is it the events of moderate magnitude and moderate frequency that are the most effective agents of erosion? Do the red paleosols developed along the wadi channels eroded in Nubian Sandstones in the desert on either side of the main Nile (Williams et al., Reference Williams, Williams and Duller2010; Williams, Reference Williams2012b) indicate a long interval of only slightly wetter climate or a shorter interval of much wetter climate? To find convincing answers to these questions we need to supplement any insights obtained from fossil soils with other independent lines of enquiry. We also need to buttress our field and laboratory observations of soil structure, texture and chemistry with additional microscopic analysis of soil fabric and mineralogy (Brewer, Reference Brewer1964; Zerboni, Reference Zerboni2005, Reference Zerboni2008; Williams et al., Reference Williams, Usai and Salvatori2015b).
Another interpretative problem may arise in the case of polygenic soil profiles. For example, in the Nubian Nile Valley of northern Sudan east of Kerma, the eastern margin of the alluvial flood plain is situated about 10–15 km from the present Nile channel. The flood plains soils are cracking clays or vertisols formed on alluvial clays laid down during the course of Holocene Nile floods, as is evident from the lenses of sub-fossil aquatic snail shells scattered throughout the parent alluvium. In some places, there are stacked layers of three or more vertisols, indicative of discrete episodes of flood plain accretion followed by cessation of flooding and the subaerial development of the cracking clay soil profiles. These are examples of polygenic soil profiles, in which soil-forming processes may continue to operate within soils of different ages. Towards the edge of the Holocene flood plain well sections reveal an alternation of alluvial sands and dark grey-brown cracking clays. In this instance, the sands are derived from local ephemeral stream channels flowing westwards from the dissected Nubian Sandstone escarpment, so that we have an inter-digitation of local wadi sands and fine gravels with distal Nile flood plain clays. The wadi sands were laid down during flash floods or intervals when the local climate was wetter than today. These sands are quite distinct from alluvial sands and gravels deposited by the Nile during times of extreme floods, which have a very different heavy mineral content, and often contain shells of the freshwater Nile mussel (Unio sp.) and the Nile oyster (Etheria elliptica), often with both valves closed and in primary context (see also Chapter 11).
4.3.5 Soils Formed on Volcanic Ash
Soils developed on volcanic ash occupy relatively small areas within the Nile Basin, including the region around Jebel Marra volcano in western Darfur, described in Chapter 13. Despite their limited extent, they are important for several reasons. First, they give rise to very fertile soils rich in potassium and other trace elements. As a consequence, they often support a dense cover of trees, herbs and grasses, providing food and shelter to mammals large and small, all of which would have been attractive for prehistoric hunters and gatherers. The soils formed on volcanic ash are not only fertile but also easy to work with simple hoes and digging sticks, and so would have been attractive to early farmers, as the terraced slopes of Jebel Marra bear witness. Finally, the ash beds would have buried and in some cases preserved some of the prehistoric plants, as in the case of the late Early to early Middle Pleistocene oil palm leaf fossils found in several piedmont localities 50 km SSW of Jebel Marra caldera (Wickens, Reference Wickens1975a, Reference Wickens1976a; Williams et al., Reference Williams, Adamson, Williams, Morton, Parry, Williams and Faure1980; Philibert et al., Reference Philibert, Tibby and Williams2010).
4.4 Conclusion
Precambrian igneous and metamorphic rocks form the underlying basement across the Nile Basin. These ancient rocks are often concealed beneath a thick mantle of mostly Palaeozoic, Mesozoic and Cenozoic sedimentary rock formations. The highlands of Ethiopia owe their origin and current elevation to uplift and volcanic activity during the last 30 Ma. The silts and clays deposited along the flood plain of the Nile in Egypt and Sudan are derived primarily from weathered volcanic rocks eroded and transported by rivers in the headwaters of the Blue Nile and Atbara drainage basins. Smaller volcanic centres are scattered across the desert region adjoining the main Nile. In the far west of the Nile Basin, Jebel Marra is a presently dormant volcano with fumaroles and hot springs that last erupted during the Holocene. It may have begun to form during the Miocene, and was intermittently active during the early to middle Pleistocene. The soils of the Nile Basin range from deep red loams developed on highly weathered rocks in the tropical south to weakly developed sandy soils formed on dunes and weathered Nubian Sandstone in the arid north. The alluvial soils flanking the Nile and its tributaries have long proved attractive to human settlement, and played a seminal role in the inception of agriculture in this region, as outlined in later chapters.