Classical chemistry and biochemistry experiments in solution measure the properties of many molecules and/or interrogate them simultaneously – these are called ensemble measurements and tend to mask the underlying molecular dynamics. Studies at single-molecule level provide random, stochastic dynamics, and allow access to an incredible wealth of molecular information. Most importantly, previously “unanswerable” questions in the physical, chemical, and biological sciences can now be answered. The field of single-molecule science (SMS) can be roughly divided into two general areas: (1) improvements in single-molecule methodologies (technology development); and (2) use of these methodologies to address important scientific questions in fundamental biological research (applied research). Over the past decades, single-molecule research has fostered excellent collaboration and interdisciplinary research with input from biology, chemistry, and physics.
Scientists are progressively aiming to discover and describe the secrets in the index of the book of life. We all know that life is a dynamic process unfolding in three dimensions, and there still remains the daunting task of fully describing the spatiotemporal location and conformation of all these indexed components, as well as their complex interactions. In order to understand such complex molecular interactions, a novel technological platform is needed. Techniques such as gene or protein chips, phage display, X-ray diffraction, nuclear magnetic resonance (NMR), and several others allow researchers to uncover part of this information; however, they lack needed sensitivity to unravel the details of individual molecular events.
1.1 The Birth of Single-Molecule Science
The initial attempt to probe individual fluorescent molecules is probably due to Jean Perrin almost a century ago (Perrin, Reference Perrin1918). However, E. A. Synge proposed an experimental scheme allowing in principle the performance of nanometer resolution microscopy in 1928, thus predating near-field optical microscopy by over 50 years. In 1948, Theodor Förster published a theoretical paper quantitatively describing the probability that light absorbed by one molecule could be transferred to another molecule and be subsequently emitted (Förster, Reference Förster1948). Possibly the first measurement of enzymatic activity at the single-molecule level was the observation by Rotman in the 1960s of fluorescent reaction products generated by a single ß-galactosidase enzyme acting on a substrate analogue (Rotman, Reference Rotman1961). In the 1970s, Hirschfeld detected a single antibody, albeit labeled with ~80 fluorophores (Hirschfeld, Reference Hirschfield1976). At IBM/Bell Labs, Arthur Ashkin had discovered optical tweezers (Ashkin, Reference Ashkin1970), a development that earned him the Nobel Prize for Physics in 2018; he found that a tightly focused laser beam could be used to trap and move micron-sized particles, but their application to biological problems took a few years. The first single-molecule measurements were first successfully performed with nonoptical methods such as the patch clamp technique, which was developed by Neher and Sakmann. This technique allowed recording of ion translocation through a single ionic channel embedded in a cell membrane (Neher and Sakmann, Reference Neher and Sakmann1976). Later on in the early 1980s, a scanning tunneling microscope was developed by Gred Binnig and Heinrich Rohrer at IBM Research, Zurich (Binnig and Rohrer, Reference Binnig and Rohrer1982), a development that also earned them the Nobel Prize for Physics in 1986. Binnig et al. (Reference Binnig, Quate and Gerber1986) described the first atomic force microscopy (AFM) in 1986. Since then, the applications of these single-molecule methods in solving biochemical and molecular biological problems have been growing rapidly.
Subsequently, few optical methods reached the sensitivity required to detect, image, manipulate, and follow the spectroscopic evolution of single fluorophores on surfaces, in solids at low temperatures (Moerner and Kador, Reference Moerner and Kador1989; Orrit and Bernard, Reference Orrit and Bernard1990). These methods also helped to detect signals directly inside live cells and to image individual DNA molecules in water (Lindsay et al., Reference Lindsay, Thundat and Nagahara1988, Reference Lindsay, Thundat and Nagahara1989; Engel, Reference Engel1991) and gliding filament and optical tweezers measurements of individual kinesin movement along microtubules (Howard et al., Reference Howard, Hudspeth and Vale1989; Block et al., Reference Block, Goldstein and Schnapp1990). The early years of the field resulted in several existing developments and improvements in a variety of fluorescence and manipulation methods, and these methods were generally applied to probe simpler chemical and biological systems. In this context, near-field scanning optical microscopy was developed, and its implementation with a simplified confocal optical microscope opened the field and made single-molecule fluorescence measurements more widely accessible and imaging was translated from low to room temperature (Betzig and Chichester, Reference Betzig and Chichester1993).
Steven Chu, who later used optical tweezers to manipulate DNA, shared the Nobel Prize in 1997 for trapping individual gas-phase molecules with the tweezers (Perkins et al., Reference Perkins, Smith and Chu1994). Then, in the 1990s, methods were also developed to observe individual molecules in solution. First, fluorescence from a single dissolved molecule (Funatsu et al., Reference Funatsu, Harada and Higuchi1997) was detected; this was quickly followed by measurement of fluorescence resonance energy transfer (FRET) between two molecules (Ha et al., Reference Ha, Enderle and Weiss1996); the observation of single-molecule events inside living cells (Mashanov et al., Reference Mashanov, Tacon and Knight2003); and recently, super-resolution in vivo imaging beyond the fundamental limit imposed by the diffraction of light waves as demonstrated by Stefan Hell (Hell, Reference Hell2007; Huang et al., Reference Huang, Babcock and Zhuang2010). In a nutshell, for this groundbreaking discovery Moerner, Betzig, and Hell were awarded the Nobel Prize in Chemistry in the year 2014, “for their work on developing super-resolution fluorescence microscopy.”
1.2 Applications of Single-Molecule Techniques in Biology
Fluorescence microscopy coupled with green fluorescent proteins brought a green revolution culminating in single fluorescent protein molecule detection. In addition, super-resolution fluorescence microscopy technique in live cells became a routine and cutting-edge tool to reveal new insights into long-standing puzzles in biology (Shashkova and Leake, Reference Shashkova and Leake2017). Single-molecule techniques are not able to provide atomic resolution pictures of large molecules such as proteins; their strengths lie in probing detailed structural distributions, as well as real-time and stochastic dynamics. Scope and applications of single-molecule science in various fields have been summarized in Figure 1.1. Few general areas will be discussed in four different parts in this volume, therefore a brief introduction is provided in this section.
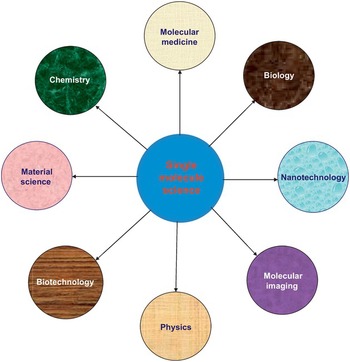
Figure 1.1 Applications of single-molecule science in various disciplines.
Protein folding: The question of how an unfolded polypeptide chain is converted into a natively folded and functional protein continues to fascinate biologists, chemists, and physicists. Conceptual breakthroughs and technological developments have flourished in this area during the past four decades. Early views of protein folding relied on theoretical descriptions developed for small-molecule reactions, involving one or a small number of specific folding pathways and intermediates (Kim and Baldwin, Reference Kim and Baldwin1982).
Biological assemblies and functions: In addition to being high-sensitivity probes of structural properties of individual biological molecules, single-molecule methods are also well suited for studying the assembly of molecular complexes and their resulting function. One important area uniquely amenable to single-molecule investigations is how conformational changes are coupled with binding. An early example came from the study of a minimal RNA junction that binds a protein S15 from the 30S small ribosomal subunit (Ha et al., Reference Ha, Zhuang and Kim1999). Ha et al. used smFRET to deduce that protein binding to the junction was accompanied by a large conformational change in the RNA junction.
Single-molecule enzymology: Soon after, Xie and coworkers reported a pioneering study of the activity of the single-molecule cholesterol oxidase enzymes that catalyze the oxidation of cholesterol by oxygen (Lu et al., Reference Lu, Xun and Xie1998), taking advantage of the intrinsically fluorescent cofactor, flavin adenine dinucleotide (FAD).
Study of motor proteins: Systems studied include the rotary F1 ATPase and synthase; cytoskeletal proteins such as kinesins, dynenins, and myosins; viral DNA-packaging motors; RNA and DNA polymerases; helicases; and chromatin-remodeling proteins (Bustamante et al., Reference Bustamante, Chemla and Forde2004; Kinosita et al., Reference Kinosita, Itoh and Yoshida2004; Mallik and Gros, Reference Mallik and Gross2004). More recently, single-molecule studies described the DNA packaging and ejection methods in greater depth (Chemla et al., Reference Chemla, Anderson and Bustmante2005; Hugel et al., Reference Hugel, Michaelis and Walter2007). Two complementary studies used single-molecule FRET on diffusing molecules and magnetic tweezers to understand mechanistic features of abortive initiation by RNA polymerase (Kapanidis et al., Reference Kapanidis, Margeat and Weiss2006; Revvakin et al., Reference Revyakin, Liu and Ebright2006), a process where as an initial transcribing complex, it undergoes several abortive cycles of short RNA synthesis and release. Several single-molecule studies have examined the activity of RNA polymerase, providing information about its binding, activity, and the transcription process in general, and a couple of recent ones are mentioned here (Zlatanova et al., Reference Zlatanova, McAllister and Leuba2006).
Study of molecular nanomachines: Molecular machines in biological cells encompass an enormous range of function, including fuel generation for chemical processes, transport of molecular components within the cell, cellular mobility, signal transduction, and the replication of the genetic code, among many others. Today, researchers are tackling the challenges of studying molecular/nanomachines in their native environments. Splicing is carried out by a cellular nanomachine called the spliceosome that is composed of RNA components and dozens of proteins. Despite decades of study, many fundamentals of the spliceosome function have remained elusive. Single-molecule detection methods enabled investigators to monitor the dynamics of specific splicing components, whole spliceosomes, and even cotranscriptional splicing within living cells (DeHaven et al., Reference DeHaven, Norden and Hoskins2016).
It is now possible to watch ribosomes in action and their structural rearrangements as they take each step necessary to transform a nucleotide sequence into a protein. Single-molecule methods were successfully applied to study protein synthesis in greater detail. A major class of single-molecule studies of protein synthesis has investigated the kinetics of the ribosome as it progresses through multiple rounds of the elongation cycle; this has been accomplished using both laser tweezers-based, single-molecule manipulation studies and fluorescence-based, single-molecule detection studies. Single-molecule studies of protein synthesis provide the dynamic information necessary to link the structural snapshots of ribosomal complexes provided by X-ray and cryo- electron microscopy structures into a real-time “movie” of how synthesis proceeds (Aitken et al., Reference Aitken, Petrov and Puglisi2010; Frank and Gonzalaz, Reference Frank and Gonzalez2010). A major class of single-molecule studies of protein synthesis is smFRET studies probing the structural dynamics of the translating ribosome. The primary objective of these studies has been to characterize the conformational dynamics that underlie mRNA-tRNA complex translocation through the ribosome. Cryo-electron microscopy is a powerful technique that could also help to unravel the secrets of ribosomal machinery and its associated protein’s conformational dynamics (Frank and Agarwal, Reference Frank and Agarwal2000).
1.3 Epigenetic Regulation
Chromatin assembly dynamics and remodeling are involved in virtually all chromosomal processes and have been the subjects of numerous single-molecule, single-fibre studies (Ladoux et al., Reference Ladoux, Quivy and Doyle2000; Brower-Toland et al., Reference Brower-Toland, Smith and Yeh2002; Zlatanova and Leuba, Reference Zlatanova and Leuba2003). Yet another recent study of remodeling enzymes by optical trapping discovered the formation of large intranucleosomal loops, which may be involved both in making nucleosomal DNA accessible and in nucleosomal mobility and eviction (Zhang et al., Reference Zhang, Smith and Grill2006). Although distinct epigenetic marks correlate with different chromatin states, how they are integrated within single nucleosomes to generate combinatorial signals remains largely unknown. Chen et al. (Reference Chen, Miller and Kirchmaier2012) have reported the successful implementation of single-molecule tools constituting fluorescence correlation spectroscopy (FCS), pulse interleave excitation-based Förster resonance energy transfer (PIE-FRET), and fluorescence lifetime imaging-based FRET (FLIM-FRET) to elucidate the composition of single nucleosomes containing histone variant H2A.Z. Quantification of epigenetic modifications in nucleosomes has provided a new dimension to epigenetics research and led to a better understanding of how these patterns contribute to the targeting of chromatin-binding proteins and chromatin structure during gene regulation.
Studies of cell surfaces using near-field nanoscopy: Living cells use surface molecules such as receptors and sensors to acquire information about and to respond to their environments. The cell surface machinery regulates many essential cellular processes, including cell adhesion, tissue development, cellular communication, inflammation, tumor metastasis, and microbial infection. These events often involve multimolecular interactions occurring on a nanometer scale and at very high molecular concentrations. Therefore, understanding how single molecules localize, assemble, and interact on the surface of living cells is an important challenge and a difficult one to address because of the lack of high-resolution single-molecule imaging techniques. However, use of AFM and near-field scanning optical microscopy (NSOM) now make it possible to provide unprecedented possibilities for mapping the distribution of single molecules on the surfaces of cells with nanometer spatial resolution, thereby shedding new light on their highly sophisticated functions.
Single molecule science, which brought a revolution in biology, can be defined as “the ability to observe and track individual molecules and monitor molecular interactions in living cells.” This new field is the resultant of fruitful collaboration among biologists, physicists, and engineers that feeds off the ability to develop new microscopes and other technology to probe single molecules and analyze their behavior in intact cells. It is quite interesting to mention that this field has emerged from developing, using, and combining technologies such as single-molecule fluorescence and super-resolution microscopy, atomic force microscopy, optical and magnetic tweezers, new sensors, and more sophisticated computing and mathematical techniques. The new frontier of single-molecule science presents us with a new way of understanding life processes differs from the bottom-up approaches. Specially, it helps to recognize the behavior and dynamics of molecules in life that give us a handle to better know the cellular architecture and molecular events (such as DNA replication, repair, transcription, translation, signal transduction, protein transport, cell division) in disease biology that ultimately helps to discover new class of drugs.
These groundbreaking discoveries paved the way for innovative techniques such as atomic force microscopy, optical tweezers, tethered particle motion, and magnetic tweezers. Additionally, fluorescence imaging (including confocal microscopy, total-internal-reflection fluorescence [TIRF], and fluorescence imaging with one nanometer accuracy techniques) has helped to visualize molecules in motion in live cells. In recent years, other imaging techniques, including super-resolution microscopies (photoactivated localization microscopy [PALM], stochastic optical reconstruction microscopy [STORM], and stimulated emission depletion [STED] microscopy), have helped biologists achieve spatial resolutions that are better than 50 nanometers (nm). Continued progress in investigating biomolecular systems of increasing complexity will be driven primarily by emerging technological breakthroughs in our abilities to observe and manipulate single molecules.
All of these studies and techniques have enabled us to study biological molecules at the single-molecule level and have led to the launch of the new field of single-molecule science or single-molecule biology.
We realized that there was the need for a book on this topic for the following reasons:
(1) In recent years, super-resolution techniques such as PALM, STORM, and STED are being applied to understand biological events at both the single-molecule and/or single-cell level, and this has generated a tremendous interest in academic research laboratories around the world in understanding their applications in molecular biology and disease biology and the potential for developing new instrumentation and diagnostic tools.
(2) Several hundred research papers have already been published in top journals, including Nature, Nature Biotechnology, Nature Methods, Nature Physics, Biophysical Journal, Science, Cell, PNAS, and many others, indicating that the use of single-molecule-based techniques in biology is growing at an exponential rate within academia, biopharmaceutical, and photonics industries.
(3) Based on this technology, many biotech companies, such as Oxford Nanpore Technologies, Ltd. UK, and Pacific Biosciences, USA, have started (using the original investigator’s proprietary patent rights) to produce novel tools, reagents, and instruments. Very recently, the large bioanalytical giant Thermo Fisher Scientific has made multimillion dollar agreements with many countries to sequence the DNA sample of human populations using single-molecule biology-based DNA sequencing. Several diagnostic companies (23 and Me, USA; Roche) are also involved in sequencing human samples for diagnostic purposes.
We have carefully selected the chapters, written by experts in the field, and have divided the chapters into appropriate parts to support the theme expressed in the subtitle of this book: From Super Resolution Microscopy, Molecular Imaging to DNA Mapping and Diagnostics. Developing novel molecular imaging tools is likely to become a prerequisite for live-image studies used to understand the inner world of the biological cells. The elegant and revolutionary single-molecule approaches that are covered in this book will undoubtedly have great future commercial promise for the development of innovative molecular image scanning procedures and for the study of biomedicine and human disease biology.
1.4 Scope of This Book
The chapters offered in the present volume discuss insights that have been revealed about their mechanism, structure, or function by single-molecule techniques. Many topics were covered in this text, such as enzymes, motor proteins, membrane channels, DNA, and other key molecules of current interest. An introduction by the editors provides a brief review of key principles with an historical overview. The last part on future perspectives discusses latest applications in relevance to molecular diagnostics and drug discovery.
The text consists of nine chapters, grouped into four parts, starting with the technology leading to descriptions of the applications, as follows:
1.4.1 Part I: Super-Resolution Microscopy and Molecular Imaging Techniques to Probe Biology
This part consists of three chapters.
In the last decade substantial improvements in the area of super-resolution techniques allowed to resolve targets in the 20 nm range, and allowed us to enable precise localization of single molecules. As Herbomel and Patterson have described in the second chapter, that the improvement of super-resolution microscopy in the last decade has led to the development of methods such as STED microscopy and structured illumination microscopy (SIM), which modulate the excitation light to break the diffraction limit, or methods such as PALM or STORM, which utilize the photo-switching properties of fluorescent molecules to enable precise localization of single molecules. Especially, this chapter focuses on PALM/STORM methods, which rely on similar principles and instrumentation for acquiring a series of images of fields of single molecules followed by subsequent image analysis to localize the molecules to much higher precision than their diffraction limited signals.
The third chapter by Gunkel et al. describes high-throughput and high-content screening, single-molecule localization microscopy, and multiscale imaging. Specially, an integration of fully automated targeted single-molecule localization microscopy (SMLM) into a screening platform is presented in order to achieve targeted microscopy and automated super-resolution imaging on tissue microarrays. This chapter also highlights on the application of total internal reflection fluorescence microscopy (TIM) microscopy to potentiate plasmid-based clustered regularly interspaced short palindromic repeats (CRISPR) usage for screening, which is the most promising high-throughput gene editing technology platform that is directly applied in human or mouse cell culture systems. DNA optical mapping, which has emerged in the 1990s as an alternative approach to optically obtain genetic and epigenetic information from single DNA molecules, allows analyzing large native genomic fragments at single-molecule resolution. Optical mapping has the potential to be an ideal tool for metagenomics studies. The use of fluorescent microscopy allows obtaining several types of information from a single molecule. In the fourth chapter, applicative studies on three different types of optical mapping-based assays, resulting in new types of data with clinical, environmental, and scientific benefits, are well presented in this book by Grunwald et al.
1.4.2 Part lI: Protein Folding, Structure, Confirmation, and Dynamics
This part consists of two chapters.
Proteins in biological systems perform a different and highly specialized role within the living cell, determined by its three-dimensional structure, composition, mechanics, and dynamics. Understanding this role is an important task for biophysicists. Very few experimental techniques are available and able to access information about the structure and dynamics of the individual elements and substructures of protein molecules. Such information is summarized in Part II. Protein nanomachines can be thought of as being built like man-made machines: static parts form a basic scaffold for movable parts whose motion is fuelled by energy source, e.g. thermal motion, ligand binding, concentration gradients or chemical reactions.
Using single molecule force spectroscopy, we are now able to identify the basic parts of these “machines”, i.e., functional mechanical elements of proteins. One such technique is to understand protein dynamics is single-molecule force spectroscopy by optical trapping, a method for which Arthur Ashkin won the Nobel Prize in Physics in 2018. Using this technique, individual conformational dynamics of protein substructures can be observed in real time, enabling a huge variety of fascinating insights into protein function to be gained. This can be seen from the example of several proteins recently studied using single-molecule force spectroscopy. In the fifth chapter, Zoldák and Tych give the details of how such high-resolution optical tweezers can be used to understand the dynamics of several proteins, including adenyl cyclase, heat shock protein 70 and 90, and AAA+ proteases.
Protein secretion is a very relevant process because more than 30 percent of synthesized proteins work in organelles or outside the cells. In Chapter 6, Wilson and his group from the University of Chile have described the posttranslational, protein translocation through membranes at the single-molecule level. His group has determined the role of essential accessory protein in translocation such as BiP and Sec 61. Although these studies allowed understanding translocation, the mechanochemistry of such transport mediated by BiP and SecA is still unknown.
1.4.3 Part III: Mapping DNA Molecules at the Single-Molecule Level
This part consists of two chapters.
Biomolecules and biopolymers undergo conformational transitions during many biological processes. For example, some proteins are observed to have multiple intermediate states in the folding/unfolding pathways, intrinsically disordered proteins can form diverse metastable structures, functional proteins can often be switched between active and inactive states through conformational transitions, and nucleosomes are able to regulate DNA unwrapping through their conformational transitions. These dynamic states of DNA and proteins control their biological functions. Since force plays a fundamental role in many, if not all, biological systems, one way to reveal the dynamics of the molecules is to elucidate its intra-and intermolecular force, which can be used as a marker to capture information about their conformational changes.
Single-molecule techniques to measure forces in these biological systems, for example, AFM, optical tweezers, and magnetic tweezers, provide a direct measurement on the force at the single-molecule level. These techniques are versatile for studying various biological processes such as ligand-receptor binding, nucleic acids unzipping, and protein folding. Therefore, in Chapter 7, Ching-Hwa Kiang’s group from Rice University has focused on using AFM in order to understand the dynamic states and pathways of conformational transitions of biological systems, thereby enabling construction of the free energy landscapes of the processes. The atomic force microscope is a key member of a series of scanning probe microscopes that allows the retrieval of local information from a surface by sensing the force operating between a specific point on the surface and a sharp probe. Atomic force microscopy, and AFM-based force spectroscopy in particular, with all the recent advancements in these techniques, is on the verge of addressing the various issues in biological systems. In Chapter 8, Joon Won Park and his colleagues from Pohang University have narrated the use of AFM to show its potential applicability as a diagnostic tool. As an example, they have summarized the use of this tool for the quantification of trace amounts of a DNA biomarker without amplification or labeling.
1.4.4 Part IV: Single-Molecule Biology to Study Gene Expression
This part consists of one chapter.
Determining rules for gene expression regulation is an important step toward predicting how cells are decoding the genome sequence to create a wide variety of phenotypes. Recent advances in imaging technologies revealed the stochastic nature of gene expression, in which different numbers of mRNA and protein molecules can be created in cells that have the same genome sequence. Characterizing and predicting heterogeneity of gene expression in single cells is a key approach to reveal mechanisms involved in stochastic gene expression, but it requires multiple fields of research. To experimentally detect stochastic behaviors of gene expression, advanced fluorescence imaging methods is essential. Especially imaging methods that have sensitivity of single mRNA or protein are crucial to measure stochasticity at any abundance, including Poissonian behaviors that emerge due to the relatively small number of mRNAs and proteins. In Chapter 9, Kumar et al. have nicely reviewed various approaches toward understanding stochastic gene expression from these multiple viewpoints.
Future Perspectives: Over the past decade, new single-cell and single-molecule analysis tools have led to advances that isolate single cells, technologies that can assay each cell's DNA, RNA, proteins, and metabolites, and imaging tools that map cell contents and their molecular interactions. These tools promise new insight on the differences in function between individual cells and molecules; the organization and timing of responses to stimuli; how cells interact as components of a complex system; and how these interactions may change with age, disease, and exposure to environmental stressors. Correspondingly, we will continue to see researchers from a variety of different disciplines make important contributions to the field in the coming years. At the molecular level, the ultimate challenge of a single-molecule experiment would be to acquire global structural time trajectories of single molecules or complexes with atomic and picoseconds resolution, while simultaneously having the ability to perturb molecular energy landscapes also at atomic resolution. There is no doubt that some of the biggest challenges and advances in single-molecule science will be in the area of live cell measurements. Current single-molecule techniques will of course continue to be used in novel and very creative ways. Single-molecule optical methods such as multiphoton fluorescence and surface-enhanced Raman scattering would complement and extend currently common technologies, such as single-molecule applications of microfluidics (Squires and Quake Reference Squires and Quake2005; Psaltis et al., Reference Psaltis, Quake and Yang2006).
This method holds promise in probing low-copy number proteins in single live cells, information not accessible by current genomic and proteomic technologies. In the last 15 years since they were first introduced, single-molecule observation and manipulation techniques have been brought to bear on the mechanisms of an ever-increasing list of biochemical reactions. In the years ahead, we expect that these methods will be applied to biomolecular systems of greater complexity. Many of the successes in studying such systems have thus far relied on the ability of the investigators to isolate, observe, and manipulate stable complexes during a relatively low-complexity segment of the catalytic cycle. An excellent example is the isolation of stable ribosomal complexes and their observation or manipulation during the elongation stage of protein synthesis. Excellent examples of dynamic assembly reactions where single-molecule studies remain challenging include (1) ribosome assembly from its constituent ribosomal RNA and ribosomal proteins components; and (2) the initiation stage of protein synthesis. Very recently, Joachim Franke and his colleagues from Columbia University, New York, USA, have adopted time-resolved cryogenic electron microscopy (TR cryo-EM) and determined the first, near-atomic resolution view of how a time-ordered series of conformational changes drive and regulate ribosomal subunits’ association and dissociation during the translation regulation, one of the most fundamental processes in molecular biology (Kaledhonker et al., Reference Kaledhonkar, Fu and Caban2019).
SMS is now a mature discipline, allowing researchers to go beyond a mere verification of established results and address unsolved questions. It will doubtless be useful in domains beyond those mentioned in this book. Its ease of implementation will entice more scientists of other disciplines to utilize its methodology, each having specific goals in mind that are difficult to foresee. Various techniques have now emerged that allow for single-molecule detection. As an example, Morisaki et al. (2016) used nascent chain tracking (NCT) to study the individual mRNA protein synthesis dynamics, and is the first time this has been achieved in vivo. Recently, researchers have made remarkable progress in the application of near-field nanoscopy to image the distribution of cell surface molecules. Those results have led to key breakthroughs: deciphering the nanoscale architecture of bacterial cell walls; understanding how cells assemble surface receptors into nanodomains and modulate their functional state; and understanding how different components of the cell membrane (lipids, proteins) assemble and communicate to confer efficient functional responses upon cell activation. We anticipate that the next steps in the evolution of single-molecule near-field nanoscopy will involve combining single-molecule imaging with single-molecule force spectroscopy to simultaneously measure the localization, elasticity, and interactions of cell surface molecules. In addition, progress in high-speed AFM should allow researchers to image single cell surface molecules at unprecedented temporal resolution. In parallel, exciting advances in the fields of photonic antennas and plasmonics may soon find applications in cell biology, enabling true nano-imaging and nano-spectroscopy of individual molecules in living cells (Dufrêne, et. al., Reference Dufrêne, Ando and Garcia2017).
Recent invention of high-speed atomic force spectroscopic techniques and automation of measurements and data analysis have pushed the boundary further. In addition, easy integration of AFM, owing to its open architecture, with other complementary techniques, including super-resolution optical microscopy, Raman and IR spectroscopy, renders it a powerful multi-dimensional platform for single-molecule studies. It is expected that continued advancements in AFM research will enrich our capabilities further, and eventually it will be possible to successfully adopt AFM-based techniques for medical diagnostics, specifically for samples where the targeted biomarkers cannot be amplified or where amplification produces significant error.
Deep Learning for Single-Molecule Science: Exploring and making predictions based on single-molecule data can be challenging, not only due to the sheer size of the datasets, but also because a priori knowledge about the signal characteristics is typically limited and because of poor signal-to-noise ratio. Although the latest development in machine learning (ML), so-called deep learning (DL), offers new avenues to address challenges, it has not been applied much in single-molecule science. (Albrecht et al., Reference Albrecht, Slabaugh and Alonso2017). Very recently, Fu et al., Reference Fu, Moonschi and Fox-Loe2019 have described a novel approach utilizing nanoscale vesicles extracted from brain regions combined with single-molecule imaging to monitor how an animal's physiological condition regulates the dynamics of protein distributions in different brain regions. This method was used to determine the effect of nicotine on the distribution of receptor stoichiometry in different mouse brain regions, and revealed that nicotine acts differentially across brain regions to alter assembly in response to exposure and withdrawal (Fu et al., Reference Fu, Moonschi and Fox-Loe2019). Since the advent of super-resolution microscopy, countless approaches and studies have been published contributing significantly to our understanding of cellular processes. With the aid of chromatin-specific fluorescence labeling techniques, we are gaining increasing insight into gene regulation and chromatin organization. Combined with super-resolution imaging and data analysis, these labeling techniques enable direct assessment not only of chromatin interactions but also of the function of specific chromatin conformational states (Birk, Reference Birk2019).
In recent years, Jennifer Doudna (University of California, Berkeley, USA) and her colleagues have used total internal reflection fluorescence microscopy (TIM) to achieve a single-molecule fluorescence visualization of CRISPR RNA-guided endonuclease Cas9 binding events (Sternberg et al., Reference Sternberg, Redding and Jinek2014). Live-cell single-molecule imaging studies have provided unique insights on how DNA-binding molecules such as transcription factors explore the nuclear environment to search for and bind to their targets. But, due to technological limitations, single-molecule experiments in living specimens have largely been limited to monolayer cell cultures. On the other hand, lattice light-sheet microscopy overcomes these limitations and has now enabled single-molecule imaging within thicker specimens such as embryos. Mir et al. (Reference Mir, Reimer and Stadler2018) have described a general procedure to perform single-molecule imaging in living Drosophila melanogaster and live mouse embryos using lattice light-sheet microscopy. This protocol allows direct observation of both transcription factor diffusion and binding dynamics. Reactions of molecules adsorbed on surfaces can be induced by injecting electrons from the tip of a scanning tunneling microscope (STM). From such an experiment, Rusimova et al. (Reference Rusimova, Purkiss and Howes2018) have concluded that picometer tip proximity regulates the lifetime of the excited state from 10 femtoseconds to less than 0.1 femtoseconds. Single-molecule bioimaging method utilizing superlocalization precision was applied toward determining snapshots of parts of the three-dimensional (3D) genome architecture and detection of conformational changes during DNA-sequence-specific binding proteins in single functional living cells (Wollman et al. (Reference Wollman, Hedlund and Shashkova2019).
On a more immediately societal level, we anticipate that single-molecule science will also have significant impact through novel biotechnologies, for example single-molecule DNA sequencing and drug discovery, and through developments in the overlapping field of nanotechnology, resulting, for example, in significant advances in individualized medicine. Finally, as the rapid progress in the field demonstrates, current techniques can be modified, improved, or extended to satisfy the requirements of the desired measurement and system under study. Meeting these challenges will ensure the continuing refinement of current techniques and the development of novel approaches. Recently, the National Academy of Sciences of USA organized a workshop (March 7–8, 2019) on the current status of this rapidly evolving field of study, reviewed the preliminary use of single-cell and single-molecule analysis tools in environmental health studies, and reported the resources needed to make the data generated most useful to the biomedical and public health fields and to regulatory decision makers.
Pros of Single-Molecule Science: Aside from observing several molecules simultaneously, single-molecule detection provides more information and benefits such as the following: (i) it allows for a molecule’s position to be accurately pinpointed within the labeled cell; (ii) information on the local dynamics and diffusion is provided by the time traces of intensity, emission spectrum, or the fluorescence lifetime; (iii) it can provide information on the proximity of specific labeled sites, less than 10 nm apart, allowing for detailed probing of reaction mechanisms; and (iv) the position sensitivity allows a scientist to locate a molecule and follow the translational motion, reorientation motion, and the internal dynamics of the individual molecules simultaneously.
Cons of Single-Molecule Science: Single-molecule detection methods do have some drawbacks: (i) the ability to detect multiple fluorescent targets simultaneously can enable visualization of complex functional and molecular processes in vivo; and (ii) in the image, one can see only a small portion of the given sample at any given time. And the higher the resolution, the worse the sampling abilities. Before using single-molecule detection techniques, one has to be cautious to study the specimen with techniques that offer less detail, but better sampling abilities.
Recently, scientists have captured high-resolution, three-dimensional images of (an enzyme in the process of precisely cutting DNA strands) gene editing enzymes in action using cryoEM, and this may help researchers develop versions of gene editing enzymes other than cryoEM that operate more efficiently and precisely to alter targeted genes and holds promise for treatment and prevention of a range of human diseases caused by DNA mutations, from cancer to cystic fibrosis and Huntington diseases. One can see how the major domains of the enzyme move during reactions, and this may be an important target for modifications.
The field of single-molecule science could also open up novel avenues for treatment by modulating or even disrupting the resilience of critical gene networks paving the way to treatments such as differentiation therapies for solid cancers.