Introduction
The arylpicolinate herbicide florpyrauxifen-benzyl (FPB) is an auxin-mimicking herbicide that belongs to Herbicide Resistance Action Committee (HRAC) Weed Science Society of America (WSSA) Group 4 (MDA 2018). Because FPB has been registered for control of postemergence weeds in freshwater aquatic sites (MDA 2018; USEPA 2017), this herbicide can be used with either foliar application to postemergence aquatic vegetation or direct application to bodies of water. Although FPB is mainly used in rice (Oryza sativa L.) with flooded irrigation systems, the herbicide also effectively controls grass and broadleaf weeds in non-flooded rice production (Wright et al. Reference Wright, Norsworthy, Roberts, Scott, Hardke and Gbur2021).
Palmer amaranth (Amaranthus palmeri S. Watson) is a threat to production of crops throughout the midsouthern United States (Norsworthy et al. Reference Norsworthy, Bangarwa, Scott, Still and Grifith2010, Reference Norsworthy, Bond and Scott2013). Since the first appearance of A. palmeri in 1989 in South Carolina (Webster and Coble Reference Webster and Coble1997), it quickly spread throughout the southern United States (Webster and Nichols Reference Webster and Nichols2012). This weed is currently listed among the most troublesome weeds that can result in the yield loss of cotton (Gossypium hirsutum L.) (6% to 65%), corn (Zea mays L.) (7% to 91%), peanut (Arachis hypogaea L.) (28% to 68%), sorghum [Sorghum bicolor (L.) Moench] (13% to 50%), sweetpotato [Ipomoea batatas (L.) Lam.](56% to 94%), and soybean [Glycine max (L.) Merr.] (17% to 79%) (Bensch et al. Reference Bensch, Horak and Peterson2003; Burke et al. Reference Burke, Schroeder, Thomas and Wilcut2007; Klingaman and Oliver Reference Klingaman and Oliver1994; MacRae et al. Reference MacRae, Culpepper, Webster, Sosnoskie and Kichler2008; Massinga et al. Reference Massinga, Currie, Horak and Boyer2001; Meyers et al. Reference Meyers, Jennings, Schultheis and Monks2010; Moore et al. Reference Moore, Murray and Westerman2004; Morgan et al. Reference Morgan, Baumann and Chandler2001; Rowland et al. Reference Rowland, Murray and Laval1999; Ward et al. Reference Ward, Webster and Steckel2013; Webster and Nichols Reference Webster and Nichols2012). Various herbicides with different sites of action have been developed to control this weed species. However, repeated use or misuse of herbicides has resulted in the evolution of herbicide resistance in A. palmeri, and the weed has consequently evolved resistance to herbicides with 10 different sites of action inhibiting auxins, acetolactate synthase (ALS), 5-enolpyruvylshikimate-3-phosphate synthase (ESPS), hydroxyphenyl pyruvate dioxygenase (HPPD), protoporphyrinogen oxidase (PPO), photosystem II Ser-264 or His-215 binders, microtubule assembly, glutamine synthetase, and very-long-chain fatty-acid (VLCFA) synthesis (Heap Reference Heap2023). A previous study has reported that pollen grains from glyphosate-resistant A. palmeri could travel to a nearby field as much as 300 m from the origin, be deposited, and pollinate susceptible A. palmeri (Sosnoskie et al. Reference Sosnoskie, Webster, Kichler, MacRae, Grey and Culpepper2012). Moreover, A. palmeri is known to have high reproductive potential, with a maximum of 600,000 seeds per plant reported (Keeley et al. Reference Keeley, Carter and Thullen1987; Ward et al. Reference Ward, Webster and Steckel2013). Thus, the resistance evolution of A. palmeri to herbicides should never be underestimated or overlooked.
Exploring mechanisms of herbicide resistance is important to obtain ideas either to attenuate the resistance in weeds or to develop herbicides with new sites of action. Two mechanisms, target-site resistance (TSR) and non–target site resistance (NTSR), are commonly evaluated to understand the causes of resistance to herbicides (Powles and Yu Reference Powles and Yu2010). TSR mechanisms, which occur primarily through genetic mutation and/or overexpression of target-site proteins, are more known than the NTSR mechanisms, which may be induced by alterations in absorption, translocation, and/or metabolism of the herbicide (Délye Reference Délye2013; Ghanizadeh and Harrington Reference Ghanizadeh and Harrington2017; Powles and Yu Reference Powles and Yu2010; Yu and Powles Reference Yu and Powles2014). However, in the case of auxin-mimicking herbicides, there are multiple potential sites of action in the auxin signaling pathway. Moreover, because numerous gene isoforms may exist in each auxin-related protein, evaluating TSR mechanisms in auxin-resistant weeds can be expensive, time-consuming, and labor-intensive.
Resistance evolution to FPB has been reported only in barnyardgrass [Echinochloa crus-galli (L.) P. Beauv.] (Hwang et al. Reference Hwang, Norsworthy, González-Torralva, Priess, Barber and Butts2021, Reference Hwang, Norsworthy, González-Torralva, Piveta, Priess, Barber and Butts2022; Jin et al. Reference Jin, Sun, Tang, Yang, Zhang, Lu and Yu2023; Takano et al. Reference Takano, Greenwalt, Ouse, Zielinski and Schmitzer2023) and not in A. palmeri. Our previous study confirmed three A. palmeri populations resistant to 2,4-D and evaluated their resistance mechanisms (Hwang et al. Reference Hwang, Norsworthy, Piveta, Souz, Barber and Butts2023). The current study investigated the presence of cross-resistance to other auxin-mimicking herbicides, such as dicamba and FPB, in the 2,4-D–resistant A. palmeri populations. Subsequently, the population with cross-resistance to FPB was subjected to resistance characterization using dose–response experiments and NTSR mechanism evaluation using the radioisotope of FPB.
Materials and Methods
Amaranthus palmeri samples
Three 2,4-D–resistant (R) A. palmeri populations were collected from Arkansas crop fields in Phillips (R1) and Lawrence (R2) counties in 2021 and Crittenden (R3) County in 2019 (Hwang et al. Reference Hwang, Norsworthy, Piveta, Souz, Barber and Butts2023). Seeds of R populations were subjected to resistance screening tests with other auxin-mimicking herbicides, such as FPB (30 g ai ha−1) and dicamba (560 g ae ha−1), registered for control of A. palmeri. The R populations were also used for metabolism inhibition experiments using metabolic inhibitors such as chloro-7-nitrobenzofurazan (NBD-Cl; 270 g ai ha−1; glutathione S-transferase [GST] inhibitor) and malathion (2,000 g ai ha−1; cytochrome P450 inhibitor) (Chen et al. Reference Chen, Wu, Wang, Yu, Bai and Pan2020; Fang et al. Reference Fang, Zhang, Liu, Yan, Li and Dong2019; Oliveira et al. Reference Oliveira, Gaines, Dayan, Patterson, Jhala and Knezevic2018; Takano et al. Reference Takano, Greenwalt, Ouse, Zielinski and Schmitzer2023). Malathion and NBD-Cl were applied 2 and 48 h before herbicide application, and the number of surviving plants was recorded 28 d after the herbicide application to calculate the mortality. Based on mechanisms by which auxin inhibitors are known to kill weeds (Grossmann Reference Grossmann2009), plants that stopped growing due to chlorosis, wilting, and necrosis were considered dead. Meanwhile, plants whose stems and leaves manifested auxin-related damage such as epinasty, swelling, curling, and cupping but kept growing with green color and new leaf production were considered alive (i.e., resistant).
Additionally, some seedlings of R populations were sprayed with 2,4-D at the labeled rate (1× rate; 1,064 g ae ha−1), and the surviving plants were grown to obtain their second filial (F2) seeds. The F2 seeds were used for subsequent dose–response and mechanism experiments. The susceptible (S) population of A. palmeri was collected from a fallow field in Miller County, AR, where the herbicides had no known history of use. In the previous screening test, the susceptible population was controlled >98% by 2,4-D (1,064 g ha−1), dicamba (560 g ha−1), FPB (30 g ha−1), glufosinate (657 g ae ha−1), glyphosate (1,263 g ae ha−1), mesotrione (105 g ai ha−1), atrazine (1,123 g ai ha−1), diuron (912 g ai ha−1), pendimethalin (1,120 g ai ha−1), fomesafen (264 g ai ha−1), and S-metolachlor (1,072 g ai ha−1).
FPB Dose–Response Experiment
The F2 generation of the R population verified with cross-resistance to FPB in the screening test (i.e., R2 population) was used for dose–response experiments. Seedlings of S and R2 populations were prepared in 50-cell plug flats filled with mediated potting soil (Sun Gro® Horticulture, Agawam, MA, USA). At the 6-leaf stage, seedlings of the S population (n = 50 plants per dose) were treated with FPB (LoyantTM, Corteva Agriscience™, Indianapolis, IN, USA) at doses of 1 to 60 g ha−1, including the labeled application rate of FPB (30 g ha−1), whereas those of the R2 population (n = 50 plants per dose) were treated with FPB doses of 2 to 480 g ha−1. One percent methylated seed oil was included in all herbicide spray solutions, and the herbicide application was performed using a track sprayer equipped with two flat-fan nozzles (1100067 TeeJet® Technologies, Springfield, IL, USA) and set at 180 L ha−1 and 1.60 km h−1. The treated plants were grown in a greenhouse with 32/22 C day/night temperatures, and the number of surviving plants was counted at 28 d after herbicide treatment based on the aforementioned criteria. Each treatment included two repetitions (i.e., 2 flats per dose). The experiment was initiated on April 15, 2023, and repeated on June 13, 2023. Percent mortality was calculated as ratios of the number of dead plants in each treatment relative to the number of tested plants per treatment (i.e., 50 plants). The dose–response experiment results were fit to the following four-parameter log-logistic model using SigmaPlot 15 (Systat Software, San Jose, CA, USA).

where y is the mortality of each A. palmeri population; C and D are the plant responses estimated at zero and infinite doses of herbicide, respectively; x and ED50 indicate the applied herbicide rate and the effective dose rate with death of 50% of treated plants, respectively; and b is the slope around x 0. The resistance-to-susceptibility (R/S) ratio of the R2 population was calculated as the ratio of ED50 values between R and S populations.
FPB Absorption and Translocation Experiment
Absorption and translocation of FPB in S and R2 A. palmeri populations were evaluated on August 14, 2023, using a phenyl ring–labeled 14C radioisotope of FPB (Corteva Agriscience™). The experiment was repeated on September 4, 2023. Seeds of each population (100 plants per population) were germinated, and 3-leaf seedlings of a uniform size were selected and transplanted to round-bottomed plastic pots (10-cm inner diameter, 9-cm height) containing the mediated potting soil. Each population included 30 pots consisting of one plant per pot. At the 6-leaf stage, FPB (30 g ha−1) was applied to the seedlings using the same application method used in the dose–response experiment. The second-youngest fully developed leaves of the applied plants were immediately treated with six droplets of [14C]-FPB solution containing 0.4 kBq radioactivity per a 0.5-μl droplet (i.e., a total of 2.4 kBq per plant). The [14C]-FPB solution was prepared in the herbicide spray solution and treated on the adaxial surface of the leaf along both sides of the plant midrib. Nontreated control plants (no [14C]-FPB) were also prepared, and all the nontreated and treated plants were grown in a growth chamber (Conviron, Winnipeg, MB, Canada) programmed at 650 µmol m−2 s−1 light intensity, 14/10 h day/night cycle, and 30/25 C day/night temperatures. Six plants were collected from each population immediately (0 h) and at 6, 12, 24, and 48 h after treatment (HAT) with [14C]-FPB. The treated leaf of plants collected was rinsed with 5 ml of methanol, after which the radioactivity in the rinsate was measured using a Tri-Carb 4910TR liquid scintillation counter (LSC; PerkinElmer, Waltham, MA, USA).
The surface of the methanol-rinsed plants was washed with tap water to remove impurities such as soil particles. The plants were cut into treated leaves, nontreated aboveground tissue, and belowground tissue. The respective tissue samples were immediately put in individual paper sacks and dried for 36 h using a freeze-dryer (Model 18DX48SA, Botanique Preservation Equipment, Phoenix, AZ, USA). The dried tissue samples were oxidized using a biological oxidizer (OX-700, R.J. Harvey Instruments, Tappan, NY, USA), and the generated 14CO2 was captured using scintillation cocktail traps. The radioactivity of 14CO2 captured was measured using the LSC. Absorption of [14C]-FPB was evaluated using percentage ratios of the sum of 14C activity observed in each plant tissue at each sampling time, relative to the sum of 14C activity measured in the rinsate and respective plant tissues at the corresponding sampling time. Translocation was calculated as percentage ratios of 14C activity measured in respective plant tissues at each sampling time, relative to the 14C activity absorbed by the plant at the corresponding sampling time.
FPB Metabolism Experiment
On the days of absorption and translocation experiments, 30 additional seedlings in the plastic pots were prepared for each of the S and R2 A. palmeri populations. Based on the same methods used in the absorption and translocation experiments, the plants were subjected to commercial herbicide application, 14C herbicide treatment, sampling, methanol rinse of the treated leaf, surface wash with tap water, and freeze-drying. The dried samples were cut to <1-cm size and transferred into a polypropylene conical tube containing 20 ml of acetonitrile/0.1 N hydrochloric acid (90:10 v/v). The samples were thoroughly homogenized using a blender (Bamix handmixer M122, ESGE, Mettlen, Switzerland), after which they were filtered through a Whatman No. 42 filter paper (GE Healthcare, Chicago, IL, USA). The filtrates were evaporated to about 0.5 ml under nitrogen blow in a 30 C water bath (XcelVap® evaporator, Biotage, Upsala, Sweden) and then reconstituted to 1 ml with acetonitrile/0.1 N hydrochloric acid (90:10 v/v). The final sample solutions were filtered using 0.2-µm PTFE syringe filters (VWR International, Radnor, PA, USA), and a 25-μl aliquot of each sample was analyzed using high-performance liquid chromatography (HPLC; Prominence-i LC-2030C 3D, Shimadzu, Kyoto, Japan) linked with a LabLogic Beta-Ram Model 4B radiation detector (RAD; LabLogic Systems, Tampa, FL, USA).
Target analytes for HPLC-RAD were two known metabolites, [14C]-florpyrauxifen-acid ([14C]-FPA; Corteva Agriscience™) and [14C]-florpyrauxifen-hydroxy acid ([14C]-FPHA; Corteva Agriscience™), along with [14C]-FPB. Total metabolism of [14C]-FPB was calculated by subtracting the [14C]-FPB analyzed at each sampling time from the 14C activity absorbed by the plant at the corresponding time. Production of metabolites was calculated as proportions of each 14C metabolite analyzed at each sampling time, relative to the 14C activity absorbed by the plant at the corresponding time.
FPA Absorption and Metabolism Experiment
Experimental methods of [14C]-FPA absorption and metabolism in S and R2 A. palmeri populations were identical to the method used in the [14C]-FPB metabolism experiment, except that the treated radioactive chemical was [14C]-FPA instead of [14C]-FPB. The experiment was initiated on September 19, 2023, and repeated on October 5, 2023. Absorption of [14C]FPA was calculated by subtracting the 14C activity analyzed in rinsate of the treated leaf at each sampling time from 14C activity analyzed in the rinsate at the initial time (0 h). Total metabolism of [14C]-FPA was calculated by subtracting the [14C]-FPA analyzed at each sampling time from the 14C activity absorbed by the plant at the corresponding time. Production of [14C]-FPHA was calculated as percentage ratios of [14C]-FPHA analyzed at each sampling time relative to the 14C activity absorbed by the plant at the corresponding time.
HPLC-RAD Analysis Conditions
Mobile phases of the HPLC system were acetonitrile and 0.1% phosphoric acid flowing at the rate of 1 ml min−1 for 26 min in a gradient mode, and the gradient recipe was the same as used in a previous study (Hwang et al. Reference Hwang, Norsworthy, González-Torralva, Priess, Barber and Butts2021; Supplementary Table S1). A reverse-phase HPLC column (ColumbusTM 5-µm C18 110 Å LC column, 250-mm long, 4.6-mm inner diameter, Phenomenex Co., Torrance, CA, USA) with a guard column (SecurityGuardTM Guard Cartridge Kit with 3.0-mm C18 column, Phenomenex) was equipped in the column oven maintained at 40 C. The flow rate of the scintillation cocktail (FlowLogic U, LabLogic Systems) for the RAD system was set at 3.0 ml min−1. Peaks of [14C]-FPB, [14C]FPA, and [14C]-FPHA appeared at 15.2 min, 11.1 min, and 6.4 min, respectively, with high specificity (Supplementary Figure S1).
Data Analysis
Predictive Analytics Software (PASW) Statistics 18 (IBM, Armonk, NY, USA) was used for all statistical analysis in the current study. A paired t-test was applied to evaluate significant differences in experimental results between S and R populations (α = 0.05). Additionally, results of time- or treatment-dependent experiments for S and R populations were subjected to ANOVA with Fisher’s LSD (α = 0.05), followed by post hoc analysis using Duncan’s multiple-range or Tukey’s honest significant difference test method (α = 0.05). Before data pooling for ANOVA, outliers among data outside the 25th to 95th percentiles based on results of box and whisker plot analysis were removed. Subsequently, data pooling was performed to estimate weighted averages from the trimmed data of each repeated experiment and then to combine results obtained from the same treatments.
Results and Discussion
Resistance Screening and Metabolism Inhibition Tests
All three 2,4-D–resistant A. palmeri populations were completely controlled by dicamba (560 g ha−1). The R3 population was also 100% controlled by FPB (30 g ha−1). The previous study concluded that the R3 population may possess TSR mechanisms to 2,4-D (Hwang et al. Reference Hwang, Norsworthy, Piveta, Souz, Barber and Butts2023). Based on the results of the current study, the 2,4-D TSR mechanism present in the R3 population did not lead to evolving cross-resistance to other auxin mimics such as dicamba and FPB. The mortality of the R1 population (98%) from FPB was similar to that of the S population (P > 0.05) (Figure 1). However, the mortality of the R2 population (65%) was significantly less than that of the S population (P < 0.05). The metabolic inhibitors NBD-Cl and malathion are known to reduce the activity of plant endogenous enzymes GST and cytochrome P450s, respectively, that can detoxify xenobiotics such as herbicides. Hence, many studies have employed such inhibitors to evaluate the presence of metabolism-based resistance mechanisms in the resistant weed populations (Chen et al. Reference Chen, Wu, Wang, Yu, Bai and Pan2020; Fang et al. Reference Fang, Zhang, Liu, Yan, Li and Dong2019; Oliveira et al. Reference Oliveira, Gaines, Dayan, Patterson, Jhala and Knezevic2018; Takano et al. Reference Takano, Greenwalt, Ouse, Zielinski and Schmitzer2023). Treatment with NBD-Cl followed by FPB did not affect the sensitivity of the R2 population to FPB. When FPB was applied following pretreatment with malathion, mortality of the R2 population increased by 15 percentage points. These results show that the FPB resistance evolved in the R2 population may be partially related to reduction in herbicide metabolism by reduced activity of cytochrome P450 enzymes. A previous study using E. crus-galli reported that the addition of malathion can keep the residual levels of the active form (FPA) higher in the weed for a longer time (Takano et al. Reference Takano, Greenwalt, Ouse, Zielinski and Schmitzer2023). The greater and longer retention of FPA by malathion may have contributed to the restoration of FPB sensitivity in some R3 plants. However, the remaining 20% of R2 plants that were not controlled by malathion pretreatment likely evolved FPB resistance by mechanisms other than enhanced metabolism, such as alterations in herbicide absorption, translocation, and/or gene sequence and expression.
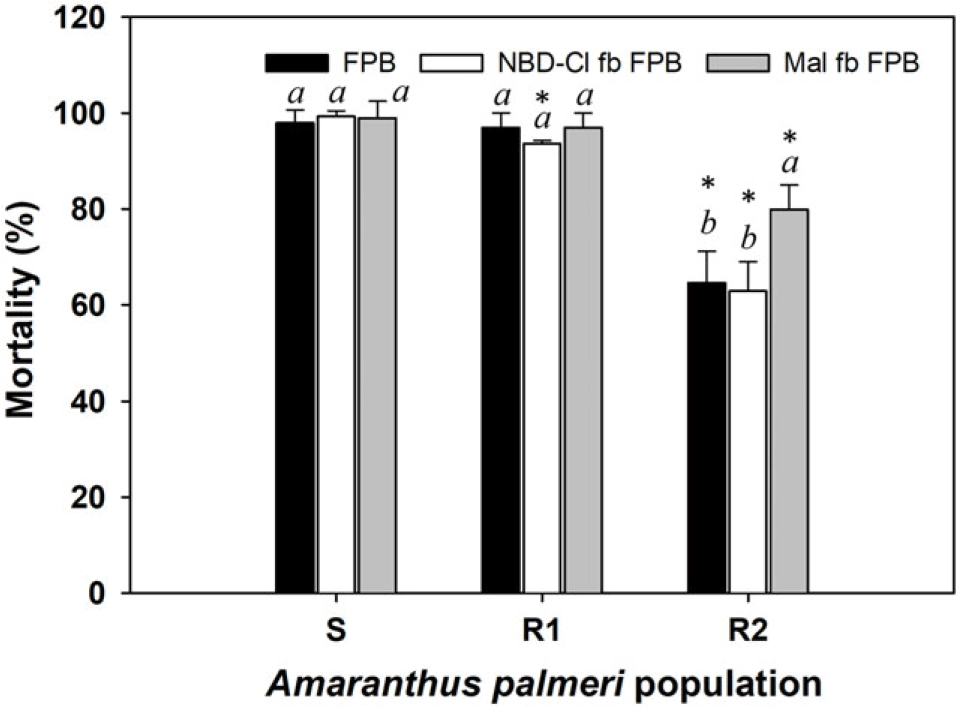
Figure 1. Mortality (%) of two 2,4-D–resistant Amaranthus palmeri populations (R1 and R2; Hwang et al. Reference Hwang, Norsworthy, Piveta, Souz, Barber and Butts2023) following application with florpyrauxifen-benzyl (FPB) alone and with chloro-7-nitrobenzofurazan (NBD-Cl) or malathion followed by florpyrauxifen-benzyl (FPB). A post hoc analysis using Tukey’s honest significant difference test method was conducted to represent differences in results in each population as influenced by treatment method (different italic lowercase letters) (P < 0.05). An asterisk (*) indicates differences in results between susceptible (S) and R populations based on a paired t-test (P < 0.05).
Dose–Response Experiment
Plants of the R2 population that survived FPB in the resistance screening test were grown to harvest seeds of the F2 generation, and the F2 seeds were used for dose–response experiments. The reason for purifying F2 seeds was to compare the resistance magnitude observed in the dose–response experiment with the results obtained later in the mechanism experiments. Because the mechanism experiments require purified F2 seeds following FPB application to enhance resistance characteristics and reduce uncertainties that may arise due to mixing with susceptible seeds, seeds for the dose–response experiment should also be the same F2 seeds as used in the mechanism experiment.
When FPB was applied at the 1× rate (30 g ha−1), the survival rate of the F2 generation (86%) was more than double that of the F1 generation (35%) (Figure 2). Unlike the S population, which was controlled completely by FPB at the 1× rate, the R2 population had plants that survived FPB at a rate eight times higher than the 1× rate (i.e., 240 g ha−1) (Figure 3). The four-parameter log-logistic model used to describe the response of the S and R2 populations to FPB fit well, with correlation coefficients of 0.99 to 1.00 (Table 1). The ED50 value of the R2 population (76.3 g ha−1) was 29-fold greater than that of the S population (2.6 g ha−1). It has been reported that A. palmeri is highly prone to evolving resistance to various types of herbicides, especially nonselective glyphosate (Culpepper et al. Reference Culpepper, Grey, Vencill, Kichler, Webster, Brown, York, Davis and Hanna2006; Molin et al. Reference Molin, Patterson and Saski2020a, Reference Molin, Yaguchi, Blenner and Saski2020b). Researchers revealed that an amplification of ESPS gene and 58 other encoding genes is responsible for evolution of glyphosate resistance in A. palmeri (Molin et al. Reference Molin, Patterson and Saski2020a, Reference Molin, Yaguchi, Blenner and Saski2020b). Under the stress of glyphosate, the transcription rates of more than 41 of these genes can be increased, and the genetic material can be transmitted to other cells in A. palmeri (Koo et al. Reference Koo, Molin, Saski, Jiang, Putta, Jugulam, Friebe and Gill2018; Molin et al. Reference Molin, Patterson and Saski2020a). During the transmission, the weed enhances the adaptation and evolution of resistance to glyphosate as well as several other herbicides with different modes of action such as auxin mimics, ALS, HPPD, PPO, and VLCFA inhibitors (Roberts and Florentine Reference Roberts and Florentine2022). FPB was registered relatively recently in 2017 (USEPA 2017). Moreover, the R2 population was collected in 2021 from an agricultural field that had not previously been sprayed with FPB. Taken together, the 29-fold reduced FPB sensitivity observed in the R2 population could likely be attributed to accumulated mechanisms of the resistance that had previously evolved to other herbicides or selection for metabolic resistance to widely different herbicide sites of action than that of FPB.
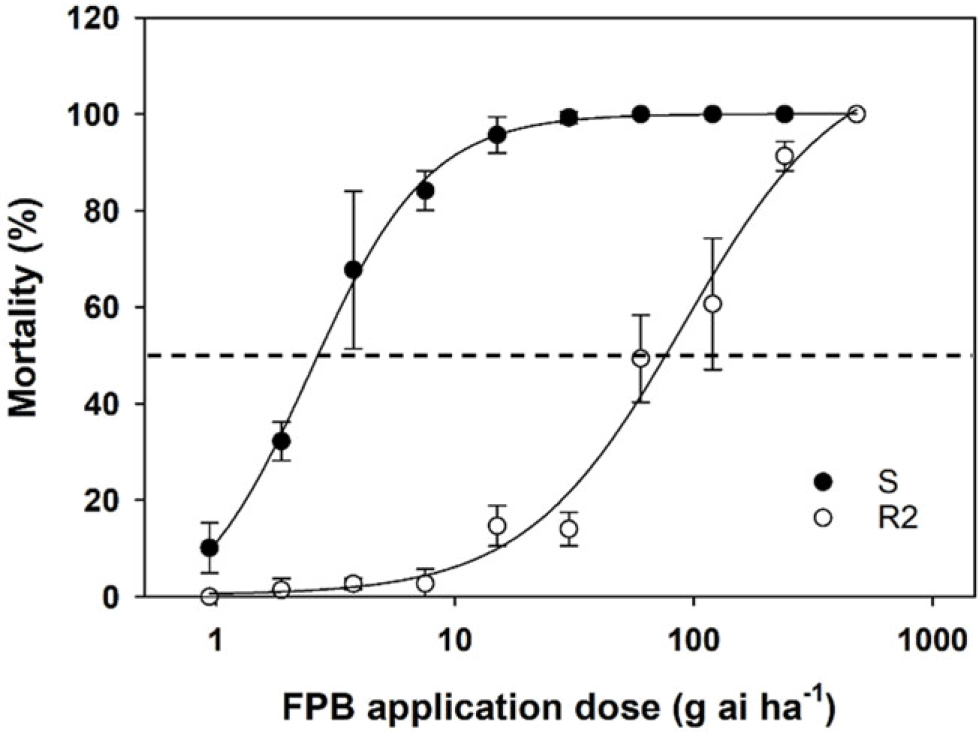
Figure 2. Four parameter log-logistic curves simulating mortality (%) of susceptible (S) and resistant (R2) Amaranthus palmeri populations 28 d after application as influenced by rates of florpyrauxifen-benzyl (FPB). The dotted line indicates the rate causing the death of 50% of tested plants (LD50), and 30 g ai ha−1 is the labeled application rate of FPB.
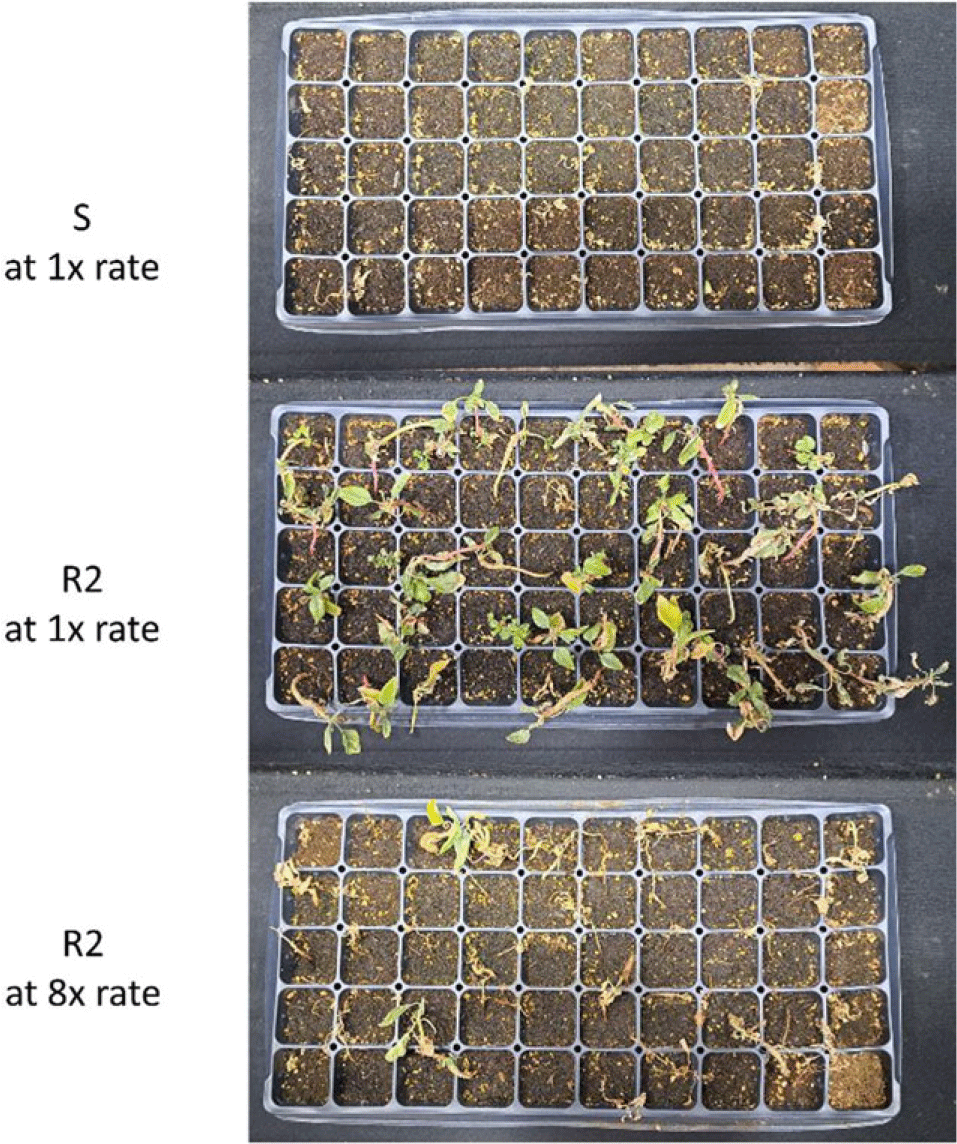
Figure 3. Representative photos of susceptible (S) and resistant (R2) Amaranthus palmeri populations 28 d following application of florpyrauxifen-benzyl (FPB) at different rates.
Table 1. Parameter values of dose–response curves based on mortality (%) results of susceptible (S) and resistant (R2) Amaranthus palmeri populations 28 d after application as influenced by rates of florpyrauxifen-benzyl (FPB).

a R2 is the correlation coefficient; ED50 is the rate giving 50% plant response.
FPB Absorption, Translocation, and Metabolism
Absorption of [14C]-FPB was similar between S (28.0% to 57.0%) and R2 (25.5% to 54.2%) populations over the entire study period (P > 0.05), showing no association of the absorption to FPB resistance evolution (Table 2). Most residues of [14C]-FPB persisted in the treated leaf (82.6% to 94.8% for the S population; 83.1% to 89.6% for the R2 population), and translocation of the residues to other plant parts was similar to or greater in the R2 population than in the S population (P ≤ 0.05). However, differences in [14C]-FPB translocation observed between S and R2 populations were too small to compare with the resistance magnitude observed in the dose–response experiment. In addition, many researchers have speculated that herbicide translocation reduced by sequestration in vacuoles of the treated leaves may be a mechanism to evolve herbicide resistance in weeds (Ghanizadeh and Harrington Reference Ghanizadeh and Harrington2017; Menendez et al. Reference Menendez, Rojano-Delgado, Prado, Myung, Satchivi, Kingston and Retention2014; Powles and Yu Reference Powles and Yu2010; Preston and Wakelin Reference Preston and Wakelin2008). Therefore, the translocation increment confirmed in this study may not be associated with the evolution of FPB resistance.
Table 2. Absorption (% of applied) and translocation (% of absorbed) of [14C]-florpyrauxifen-benzyl (FPB) in susceptible (S) and resistant (R2) Amaranthus palmeri populations at 6, 12, 24, and 48 h after treatment (HAT).
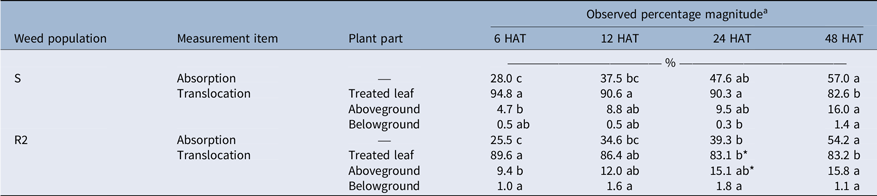
a A post hoc analysis using Duncan’s multiple-range test method was conducted to represent differences between results observed at different sampling times (different lowercase letters) (P < 0.05). An asterisk (*) indicates differences in results between S and R2 populations based on a paired t-test (P < 0.05).
More than 98% of [14C]-FPB residues absorbed by S and R2 A. palmeri populations were metabolized within 6 h, and the metabolism magnitude was maintained over the 48-h study period (Figure 4). The 6-h metabolism observed in the tested A. palmeri was greater than the 24-h metabolism observed for E. crus-galli in a previous study (82% to 89%; Hwang et al. Reference Hwang, Norsworthy, González-Torralva, Priess, Barber and Butts2021). Although the total metabolism was similar between S and R2 populations throughout the study period, production of [14C]-FPA for 24 h after herbicide application was greater in the S population than in the R2 population (P < 0.05). Similar to results of previous studies that investigated mechanisms of FPB resistance in E. crus-galli (Hwang et al. Reference Hwang, Norsworthy, González-Torralva, Priess, Barber and Butts2021, Reference Hwang, Norsworthy, González-Torralva, Piveta, Priess, Barber and Butts2022; Takano et al. Reference Takano, Greenwalt, Ouse, Zielinski and Schmitzer2023), the reduced production and/or rapid degradation of FPA might be one of the mechanisms that A. palmeri evolved resistance to FPB. However, given that FPB sensitivity was 29-fold lower in the R2 population than in the S population, the difference in [14C]-FPA residues between S and R2 populations does not seem to be enough to account for the FPB resistance mechanisms. In the results of the [14C]-FPHA analysis, no significant differences were found between S and R2 populations. Further studies would be needed to investigate the presence of TSR in the R2 population.
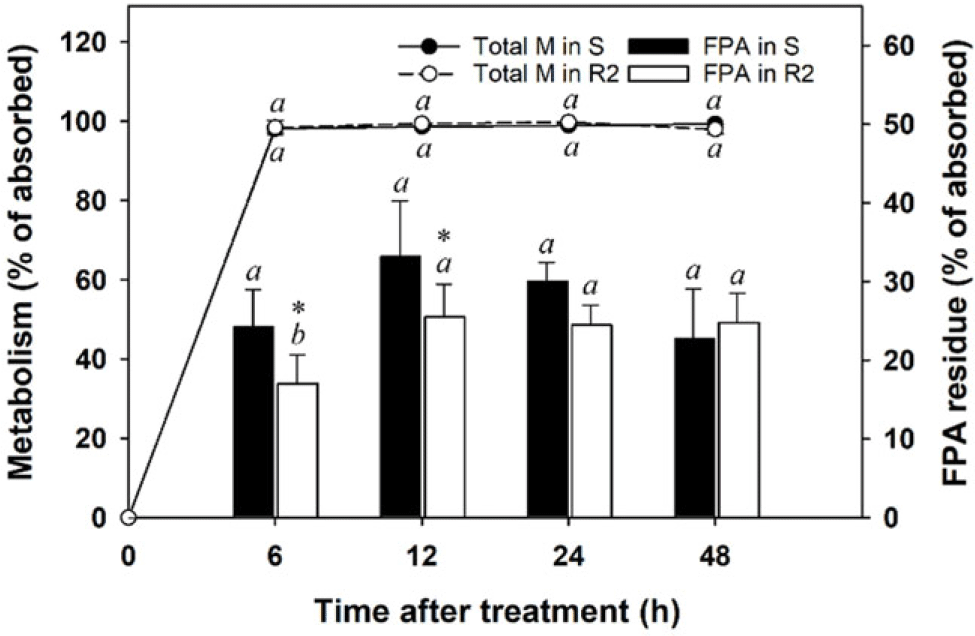
Figure 4. Metabolism of [14C]-florpyrauxifen-benzyl (FPB) and its convention to [14C]-florpyrauxifen-acid (FPA) in susceptible (S) and resistant (R2) Amaranthus palmeri populations. Error bars represent standard deviations (n = 6). A post hoc analysis using Duncan’s multiple-range test method was conducted to represent differences in results in each population at the different sampling times (different italic lowercase letters) (P < 0.05). The asterisk (*) indicates differences in results between S and R2 populations based on a paired t-test (P < 0.05).
FPA Absorption and Metabolism
Absorption and metabolism experiments following the application of [14C]-FPA were conducted to verify whether smaller detection of [14C]-FPA in the R2 population after [14C]-FPB application was due to the reduced production of [14C]-FPA or to its rapid degradation. Foliar absorption of [14C]-FPA was maximized in both S and R2 populations 6 h after application and was similar for R2 (94.1% to 97.0%) and S (93.8% to 98.0%) populations over the entire experiment period (P > 0.05; Figure 5). The metabolism of [14C]-FPA absorbed by the tested A. palmeri populations increased consistently over time and was greater in the R population (45.1% to 72.5%) than in the S population (27.0% to 64.3%) (P < 0.05). The enhanced FPA metabolism in the R2 population likely contributed to FPB resistance being conferred to A. palmeri.
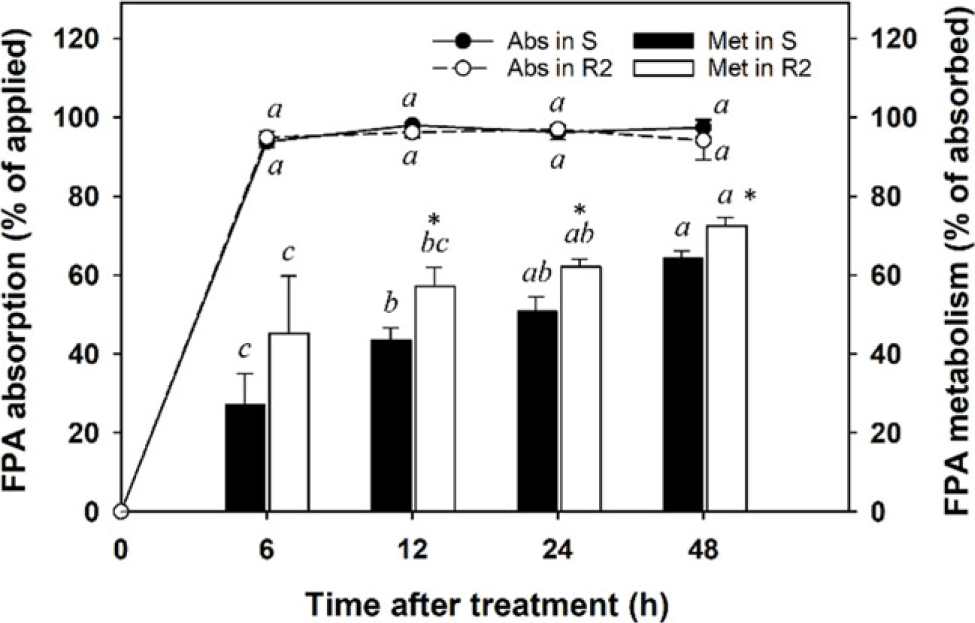
Figure 5. Absorption and metabolism of [14C]-florpyrauxifen-acid (FPA) in susceptible (S) and resistant (R2) Amaranthus palmeri populations following [14C]-FPA treatment. Error bars represent standard deviations (n = 6). A post hoc analysis using Duncan’s multiple-range test method was conducted to represent differences in results in each population at the different sampling times (different italic lowercase letters) (P < 0.05). An asterisk (*) indicates differences in results between S and R2 populations based on a paired t-test (P < 0.05).
The analytical results of the final metabolite [14C]-FPHA produced after [14C]-FPA application also prove the enhanced metabolism of [14C]-FPA in the R2 population (Table 3). The production of [14C]-FPHA increased in all S and R populations over time and was overall greater in the R population (4.8% to 21.4%) than in the S population (3.3% to 12.3%). The greater production of [14C]-FPHA in the R population was likely related to the enhanced [14C]-FPA metabolism in that population. Taken together, in the prior experiment conducted after the application of [14C]-FPB, the reduced detection of [14C]-FPA in the R population might also be attributed to the enhanced metabolism of [14C]-FPA in that R population. However, the possibility remains that the conversion of [14C]-FPB to [14C]-FPA may have been reduced in the R2 population.
Table 3. Production (% of absorbed) of [14C]-florpyrauxifen-hydroxy acid (FPHA) in susceptible (S) and resistant (R2) Amaranthus palmeri populations at 6, 12, 24, 48 h after [14C]-florpyrauxifen-acid (FPA) treatment (HAT).
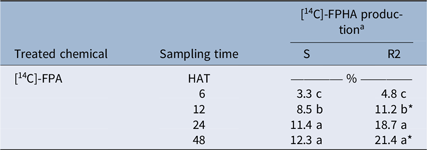
a A post hoc analysis using Duncan’s multiple-range test method was conducted to represent differences between results observed at different sampling times (different lowercase letters) (P < 0.05). An asterisk (*) indicates differences in results between S and R2 populations based on a paired t-test (P < 0.05).
The current study confirmed that one of three A. palmeri populations verified with resistance to the auxin-mimicking herbicide 2,4-D in a previous study (i.e., R2 population) exhibits simultaneous resistance to another auxin-mimicking herbicide, FPB. Because the R2 population has not been previously exposed to FPB, the FPB resistance in that population is likely due to the accumulated mechanisms of resistance that have evolved to other herbicides with the same and/or different modes of action. Given the cross-resistance case of A. palmeri to 2,4-D and FPB, growers should not alternately use different herbicide actives with the same mode of action, but should use herbicides having different modes of action in rotation or mixture. The FPB resistance could be partially influenced by enhanced activity of the plant detoxification enzyme cytochrome P450s, but NTSR mechanisms such as FPB absorption, translocation, and total metabolism were not associated with the evolution of FPB resistance in the R2 population. However, less retention of the active acid metabolite FPA was observed in the R2 population than in the S population, which shows that the rapid metabolism and reduced production of FPA may partially contribute to endowing FPB resistance to the R2 A. palmeri population. Because the NTSR evaluation results obtained in the current study are insufficient to account for the 29-fold reduced FPB sensitivity in the R2 population, further genetic studies are needed to evaluate the presence of TSR mechanisms in the R2 population. The results obtained in this study may be useful for weed scientists and herbicide developers seeking an understanding of the mechanisms of evolution of cross-resistance to auxin herbicides in A. palmeri and alternatives to mitigate the resistance or insights to develop new herbicide actives.
Supplementary material
To view supplementary material for this article, please visit https://doi.org/10.1017/wsc.2023.72
Acknowledgments
Corteva Agriscience™ provided radiolabeled florpyrauxifen-benzyl, florpyrauxifen-acid, and florpyrauxifen-hydroxy acid. The Arkansas Rice Research and Promotion Board partially supported this project. The authors declare no conflict of interest.