The evolution of tetrapod limbs from fish fins is one of the best-studied transitions in vertebrate evolution (Clack Reference Clack2005, Reference Clack2009). In the quest for unravelling this key evolutionary event, much attention has been placed on detailing anatomical modifications in taxa bracketing the transition (e.g., Coates et al. Reference Coates, Ruta and Friedman2008; Clack Reference Clack2009; Diogo et al. Reference Diogo, Johnston, Molnar and Esteve-Altava2016; Ruta & Willis Reference Ruta and Wills2016; Molnar et al. Reference Molnar, Diogo, Hutchinson and Pierce2017), as well as determining the developmental and genetic basis of limb (and digit) formation (e.g., Shubin & Alberch Reference Shubin, Alberch, Hecht, Wallace and Prance1986; Johanson et al. Reference Johanson, Joss, Boisvert, Ericsson, Sutija and Ahlberg2007; Cole et al. Reference Cole, Hall, Don, Berger, Boisvert, Neyt, Ericsson, Joss, Gurevich and Currie2011; Boisvert et al. Reference Boisvert, Joss and Ahlberg2013; Schneider & Shubin Reference Schneider and Shubin2013; Gehrke et al. Reference Gehrke, Schneider, de la Calle-Mustienes, Tena, Gomez-Marin, Chandran, Nakamura, Braasch, Postlethwait, Gómez-Skarmeta and Shubin2015; Zuniga Reference Zuniga2015; Nakamura et al. Reference Nakamura, Gehrke, Lemberg, Szymaszek and Shubin2016). A further area of exploration has focused on understanding the biomechanical implications of limbs versus fins and how tetrapods made the ecological ‘leap' from living in water to walking on land (e.g., Edwards Reference Edwards, Hecht, Goody and Hecht1977; Fricke et al. Reference Fricke, Reinicke, Hofer and Nachtigall1987; Pridmore Reference Pridmore1994; King et al. Reference King, Shubin, Coates and Hale2011; Pierce et al. Reference Pierce, Clack and Hutchinson2012; Kawano & Blob Reference Kawano and Blob2013; Pierce et al. Reference Pierce, Hutchinson and Clack2013). Although much progress has been made in understanding how tetrapod limbs may have originated, we know very little about either why limbs evolved or the impetus for tetrapods to become terrestrial-going animals.
In the book Gaining ground, Clack (Reference Clack2012) provides a comprehensive synthesis of the various lines of evidence that give us a picture of the origin and evolution of tetrapods. Within, theories for why tetrapods (and their limbs) evolved are outlined. Historically, the drying-up-pool hypothesis was favoured, in which stranded fish (e.g., Eusthenopteron) were under selective pressure to evolve more limb-like appendages to return to water (e.g., Romer Reference Romer1958). But this ‘terrestriality before limbs' scenario has generally fallen by the wayside (Clack Reference Clack2012; Pierce et al. Reference Pierce, Hutchinson and Clack2013); one reason is that it hinged on the notion that the Devonian red beds were deposited in arid conditions, when such geological signatures can also correlate with wet environments. In light of new fossil evidence pointing towards ‘limbs before terrestriality', more recent ideas range from station-holding, to spreading body mass load in partially submerged animals, and even for propping the head out of water to breathe air (Coates & Clack Reference Coates and Clack1995; Clack Reference Clack2002, Reference Clack2007, Reference Clack2009; Shubin et al. Reference Shubin, Daeschler and Jenkins2006.). Of course, there may have been multiple, interconnected selective pressures driving limb (and digit) evolution.
Beyond the fossil record, insight into tetrapod limb evolution may be gained from studying fishes. Limb-like adaptations have repeatedly evolved in fishes that regularly interact with substrate – whether submerged in water or emersed on land – and many engage in ‘walking' behaviours (Renous et al. Reference Renous, Davenport and Bels2011; Pierce et al. Reference Pierce, Hutchinson and Clack2013). Recently, there has been a flurry of research detailing how fishes utilise their fins for substrate driven locomotion. For example, fin anatomy and locomotor patterns have been described in walking sharks and rays (Pridmore Reference Pridmore1994; Goto et al. Reference Goto, Nishida and Nakaya1999; Lucifora & Vassallo Reference Lucifora and Vassallo2002; Macesic et al. Reference Macesic, Mulvaney and Blevins2013), land-traversing catfish (Johnels Reference Johnels1957; Gougnard & Vandewalle Reference Gougnard and Vandewalle1980; Pace Reference Pace2009), crutching mudskippers (Harris Reference Harris1960; Pace & Gibb Reference Pace and Gibb2009; Kawano & Blob Reference Kawano and Blob2013), pectoral-fin-walking bichirs (Standen et al. Reference Standen, Du and Larsson2014, Reference Standen, Du, Laroche and Larsson2016; Wilhelm et al. Reference Wilhelm, Du, Standen and Larsson2015; Foster et al. Reference Foster, Dhuper and Standen2018), pelvic-fin-walking lungfish (King et al. Reference King, Shubin, Coates and Hale2011; Aiello et al. Reference Aiello, King and Hale2014; King & Hale Reference King and Hale2014), and ambulatory cavefish (Flammang et al. Reference Flammang, Suvarnaraksha, Markiewicz and Soares2016). One of the best underwater ‘walkers' – the frogfishes (Antennariidae) – are often alluded to in the context of tetrapod evolution, as their fins are perhaps the closest analogue to tetrapod-digited limbs (Edwards Reference Edwards1989; Clack Reference Clack2012).
Frogfishes are nested within a paraphyletic grade of bottom-dwelling anglerfishes (Teleostei: Lophiiformes; Fig. 1) that, together, are related to a clade of deep-sea pelagic swimmers (Ceratioidei; Miya et al. Reference Miya, Pietsch, Orr, Arnold, Satoh, Shedlock, Ho, Shimazaki, Yabe and Nishida2010). A uniting feature of anglerfishes is their illicium or lure that grows out of their head and is used to attract prey (Pietsch & Grobecker Reference Pietsch and Grobecker1987). Frogfishes live at various water depths and use their pediculate (‘like little feet') fins to ‘walk' along the substrate, navigate through rocks or over corals, or clamber about in seaweed (Lindsey Reference Lindsey, Hoar and Randall1978; Pietsch & Grobecker Reference Pietsch and Grobecker1987). Qualitative analysis of fin-fall patterns has shown frogfishes use at least two locomotory behaviours: the first is a crutching-like motion similar to mudskippers (although under water it is more of a gallop) where the large pectoral fins generate thrust and the small pelvic fins stabilise the body during the recovery stroke; the second involves alternating movements of all four fins – similar to a tetrapod lateral-sequence gait (Pietsch & Grobecker Reference Pietsch and Grobecker1987; Edwards Reference Edwards1989; Renous et al. Reference Renous, Davenport and Bels2011).
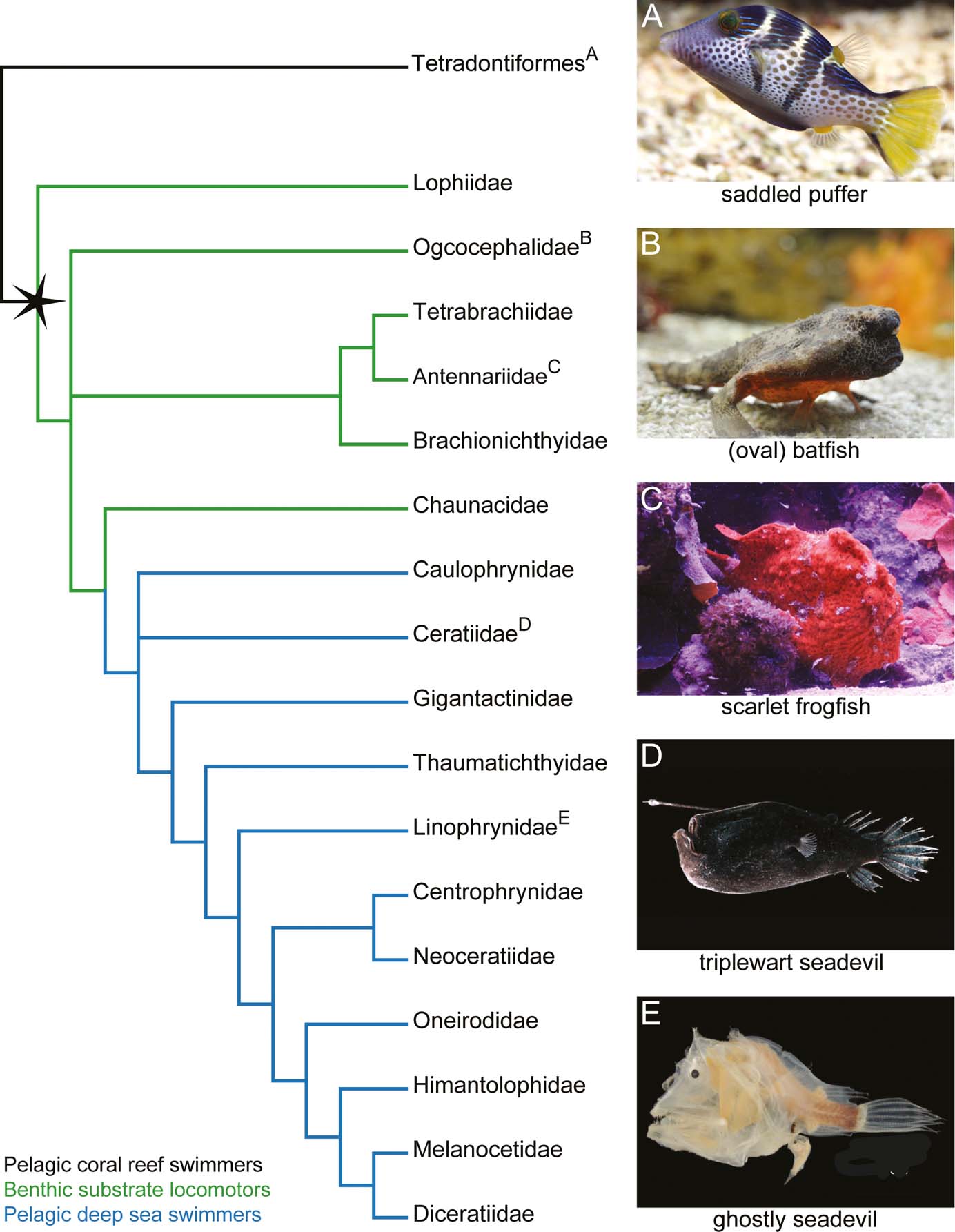
Figure 1 Family-level evolutionary relationships of the Lophiiformes based on molecular data (Miya et al. Reference Miya, Pietsch, Orr, Arnold, Satoh, Shedlock, Ho, Shimazaki, Yabe and Nishida2010) and life photos of the anglerfish species examined in this study. (A) Saddle puffer (Canthigaster valentine), an example of an outgroup tetrapodontiform (photo by Karelj released into the public domain). (B) Representative batfish (Ogcocephalus nasutus), as no appropriate photo exists of the oval batfish (Ogcocephalus notatus; photo by Gilles San Martin/CC BY). (C) Scarlet frogfish (Antennarius coccineus) (photo by Robert Wielgorski/GNU FDL). (D) Triplewart seadevil (Cryptopsaras couesii) (photo by Edith Widder/CC BY). (E) Ghostly seadevil (Haplophryne mollis) (photo by Museum of Comparative Zoology, Harvard/CC BY).
Exactly how the fins of frogfishes have been modified to behave like limbs is not well established. Monod (Reference Monod1960) provides the most thorough description of frogfish/anglerfish pectoral fin musculoskeletal structure. The two-dimensional (2D) illustrations of the associated dissections offer great insight into fin anatomy, but no quantitative data is presented and there has been a lack of confirmatory observations. Here, we use contrast-enhanced micro-computed tomography (μCT) scanning to investigate the three-dimensional (3D) anatomy of both the soft and hard tissues of the pectoral fin in a representative frogfish – the scarlet frogfish (Fig. 1c). The musculoskeletal data are compared to three additional anglerfishes, including: the benthic ‘walking' oval batfish (Ogcocephalidae; Fig. 1b), which represents an independent acquisition of fin-assisted walking; and two deep-sea pelagic seadevils – the triplewart seadevil (Ceratiidae; Fig. 1d) and the ghostly seadevil (Linophrynidae; Fig. 1e). Through this comparative lens, we discuss potential adaptations of the limb-like pectoral fins in frogfishes (and batfishes), as well as anatomical trade-offs that may enable diverse types of fin-assisted locomotor behaviours.
1. Material and methods
1.1. Species and specimens
We examined four species of anglerfish: two species of deep-sea pelagic swimmers and two species of benthic substrate locomotors (Figs 1, 2). Species and specimens were chosen primarily based on their locomotor ecology, completeness, and commonality in the Museum of Comparative Zoology (MCZ) Ichthyology Collection. Specimens were also chosen by their ability to be contrast-enhanced stained and μCT scanned in a reasonable timeframe, as the largest specimens of some anglerfish species can grow too large to efficiently stain or scan using a standard μCT scanner. The pelagic species include the triplewart seadevil, Cryptopsaras couesii (MCZ:Ich:76459; Fig. 2a), and the ghostly seadevil, Haplophryne mollis (MCZ:Ich:161516; Fig. 2b). Both are bathypelagic swimmers and are found globally in deep waters at depths as great as 2000m (Froese & Pauly Reference Froese and Pauly2018). The two benthic species include the scarlet frogfish, Antennarius coccineus (MCZ:Ich:6807; Fig. 2c), and the oval batfish, Ogcocephalus notatus (MCZ:Ich:45075; Fig. 2d). The scarlet frogfish is a benthic reef-dwelling fish that reaches sizes of up to 91mm; it is found largely in the tropical Indo-Pacific around Australia and in the waters of East Africa, the Red Sea, and California, at depths of up to 75m (Pietsch & Grobecker Reference Pietsch and Grobecker1987). The oval batfish is also benthic but inhabits more open seabeds rather than reefs and can grow up to 130mm; it is found in tropical waters of the West Atlantic and Caribbean Sea at depths of 15–172m (Froese & Pauly Reference Froese and Pauly2018). Compared to the frogfishes, which have round bodies and are inefficient swimmers, batfishes are dorsoventrally compressed and can swim in short bursts. Both frogfish and batfish use their fins to ‘walk' around their habitat.
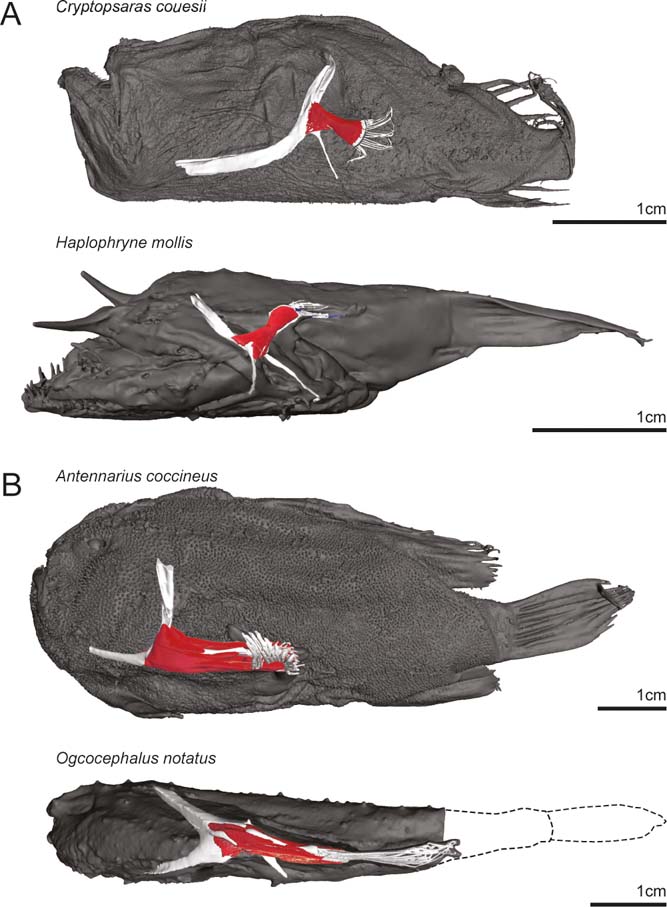
Figure 2 Full-body digital reconstructions with segmented pectoral girdles and fins overlaid. (A) Pelagic deep-sea swimming species Cryptopsaras couesii (triplewart seadevil) and Haplophryne mollis (ghostly seadevil). (B) Benthic substrate locomotor species Antennarius coccineus (scarlet frogfish) and Ogcocephalus notatus (oval batfish).
1.2. Tissue staining, μCT scanning, and segmentation
To visualise the 3D morphology of the muscles and skeleton of the pectoral fin, whole alcohol-preserved specimens were stained to enhance soft tissue contrast with 2.5 % w/v phosphomolybdic acid (PMA) in 70 % ethanol for up to 21 days. Of all the common contrast agents available, iodine (I2KI) being the most common, PMA provides the best discrimination of all soft tissues, including cartilage (Descamps et al. Reference Descamps, Sochacka, De Kegel, Van Loo, Van Hoorebeke and Adriaens2014). In order to minimise overstaining, specimens were scanned at intervals during the staining processes to track penetration of the stain. All specimens were scanned in the MCZ using a Bruker SkyScan 1173 (for settings, see Table 1). Once tissues of the pectoral fin were fully penetrated by the contrast stain, the bones, cartilage, and individual muscles were digitally dissected by manual segmentation using Materialise Mimics®, and identified following the text and diagrams of Monod (Reference Monod1960). The bones/areas of muscle attachment at the origin and insertion were also identified.
Table 1 Species, specimen numbers, μCT scan settings, and days immersed in contrast-enhanced stain. Abbreviations: MCZ = Museum of Comparative Zoology, Harvard University; Al = aluminium.

1.3. Individual muscle properties
Using Materialise Mimics®, two muscle properties were digitally measured on each muscle: muscle volume and fibre length. Muscle volume was recorded directly from each segmented mesh. Fibre lengths were determined by using the measure distance tool on three separate fibres for each muscle and taking the average. Physiological cross-sectional area (PCSA) was then calculated for each muscle as:

The specific tension of lophiiform muscle is currently unknown. Though data is available for other fish species, values range from 65kNm–2 in European eel (Ellerby et al. Reference Ellerby, Spierts and Altringham2001) to 289kNm–2 in dogfish shark (Lou et al. Reference Lou, Curtin and Woledge2002). As force is proportional to PCSA, we use PCSA as a proxy for force capacity. All muscle properties were normalised to body mass (M b) to allow comparison between species of different sizes. Using geometric similarity, volumes were divided by M b; fibre lengths were divided by M b1/3; and PCSAs were divided by M b2/3.
In addition to comparing muscle properties across the four species sampled, we generated a functional morphospace by plotting normalised PCSAs against normalised fibre lengths. As PCSA is an indicator of force and fibre length is proportional to the range of muscle shorting (or distance over which force can be generated), a functional morphospace provides a visualisation of the physiological trade-offs within and between muscles (Lieber Reference Lieber2002).
1.4. Coordinate system
The species examined here all hold their pectoral fins in different orientations relative to the main horizontal axis of the body (Fig. 2). The pectoral fin of Cryptopsaras is held flat against the body with the ‘adductor' surface facing the body and is directed posteriorly. In Haplophryne the pectoral fin is also held flat against the body but is instead directed posterodorsally. The pectoral fin in Antennarius is directed posteriorly and has been pronated slightly such that the ‘adductor' surface faces more dorsally. Compared to Antennarius, the pectoral fin of Ogcocephalus is even further modified, with both the girdle being dorsoventrally compressed and the fin projecting more laterally from the body. The fin of Ogcocephalus is pronated even further than Antennarius such that the ‘adductor' surface faces dorsally.
To effectively compare different bones, joints, and muscle actions between pectoral fins that are oriented differently with respect to the body, we established a coordinate system. Here the glenoid is treated as a three-degree-of-freedom joint with the following actions: protraction/retraction is anterior/posterior movements along the horizontal plane of the body; elevation/depression is dorsal/ventral movements around the horizontal plane of the body; and pronation/supination is inward/outward rotation around the long axis of the fin. We also describe flexion/extension of the lepidotrichia as follows: flexion is movement of the lepidotrichia towards the ‘abductor' surface of the fin (or towards the substrate in benthic species) and extension is movement of the lepidotrichia towards the ‘adductor' surface of the fin (or away from the substrate in benthic species).
2. Results
2.1. Skeletal morphology
The pectoral fin is relatively larger and longer in benthic species as compared to pelagic species (Fig. 2). In particular, Antennarius and Ogcocephalus have longer and more robust radials and lepidotrichia. The larger radials have the effect of increasing the overall bony area for muscle attachment.
2.1.1. Shoulder girdle
In all species, the shoulder girdle is a highly integrated structure of cleithral bones and the scapulocoracoid. Sutures delimiting the various bones were very subtle in the scans, making it almost impossible to segment out each component part; thus, we describe the shoulder girdle as a whole complex. The shoulder girdle of Cryptopsaras is mediolaterally flattened, with a long and ventrally projecting process extending from the coracoid (Figs 2a, 3a). In Haplophryne (Figs 2b, 3a), the girdle is more gracile compared to Cryptopsaras and has been rotated forward with the metacleithrum (syn. postcleithrum) curving under the body. The shoulder girdle of Antennarius (Figs 2c, 3b) is more robust with the dorsal process of the cleithrum pointing dorsally and the anterior process pointing anteromedially; the metacleithrum is either reduced or is altogether absent. The cleithrum in Ogcocephalus (Figs 2d, 3b) is dorsoventrally flattened and rotated forward, with each process angled more medially; it also bears a robust posterior-facing metacleithrum. Both Antennarius and Ogcocephalus have a coracoid process that forms a strut between the cleithrum and coracoid and provides a surface for muscle attachment (Figs 3b, 4).
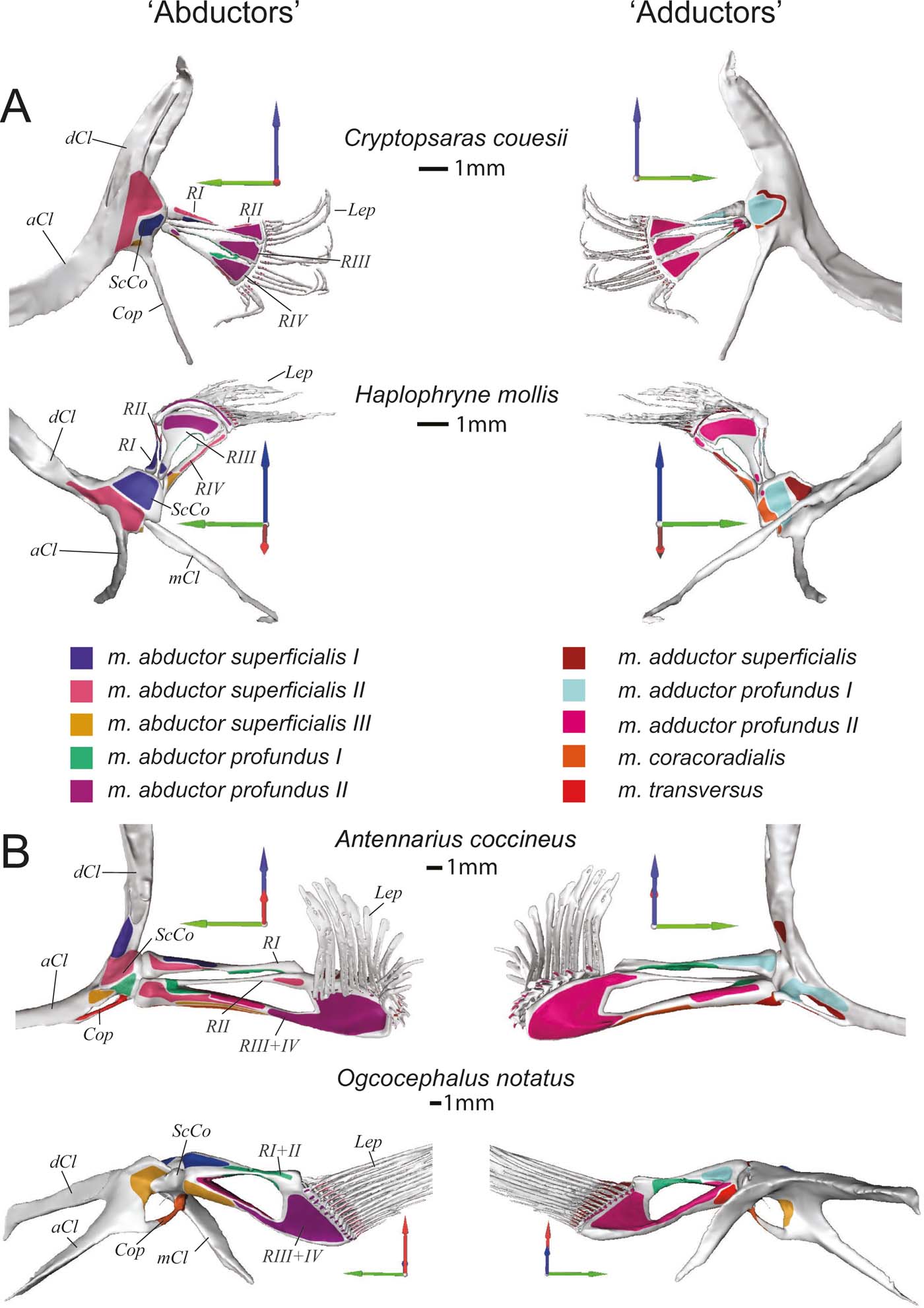
Figure 3 Skeletal morphology of the pectoral fin and girdle showing muscle origins and insertions from the ‘abductor' (left) and ‘adductor' (right) surfaces. (A) Pelagic species Cryptopsaras couesii and Haplophryne mollis. (B) Benthic species Antennarius coccineus and Ogcocephalus notatus. Joint coordinate system: blue = dorsal; green = anterior; red = lateral. Abbreviations: aCl = anterior process of cleithrum; Cop = coracoid process; dCL = dorsal process of cleithrum; Lep = lepidotrichia; mCl = metacleithrum; ScCo = scapulocoracoid; RI–IV = radials I–IV. Origins and insertions are colour coded for homology.

Figure 4 Comparison of glenoid joint morphology across the four anglerfish species studied. (A) ‘Abductor' view, with joints disarticulated to visualise joint morphology. (B) Radials reflected to visualise articular surface. Note the reverse ball-and-socket joint of Antennarius and Ogcocephalus. Dashed line indicates the approximate suture between the cleithrum and scapulocoracoid (which is not identifiable in Ogcocephalus). Abbreviations: Cl = cleithrum; Cop = coracoid process; Lep = lepidotrichia; mCl = metacleithrum; ScCo = scapulocoracoid; RI–IV = radials I–IV.
The glenoid joint is formed by the scapulocoracoid and radials and has been modified to varying degrees (Fig. 4). In Haplophryne, the glenoid has no clear bony or cartilaginous guiding structure, but Cryptopsaras has a more developed glenoid, where the radials come together to form a slight rounding. The radials in Antennarius and Ogcocephalus are proximally integrated and form a pronounced concavity, which articulates with the scapulocoracoid in a reverse ball-and-socket glenoid joint.
2.1.2. Radials
Both pelagic species have four radials (Figs 3a, 4), labelled I–IV from dorsal to ventral, the dorsal-most radial (radial 0) having been lost (Monod Reference Monod1960). In contrast, the benthic species have reduced their radials to three in Antennarius and two in Ogcocephalus through fusion (Figs 3b, 4). In both Antennarius and Ogcocephalus, radials III and IV are fused into the bone we refer to as radial III+IV. Radials I and II are further fused in Ogcocephalus into the radial we refer to as radial I+II. Regardless of fusion state, the radials articulate proximally with the scapulocoracoid at the glenoid joint in all species (Fig. 4). The radials articulate distally with the lepidotrichia via the cartilaginous epactinal rod (Monod Reference Monod1960; Edwards Reference Edwards1989) – interpreted as a series of fused distal radials (Consi et al. Reference Consi, Seifert, Triantafyllou and Edelman2001). The epactinal rod is likely present in all species, though due to limitations in scan resolution and contrast we were only able to segment it in Antennarius and Ogcocephalus (Fig. 5).
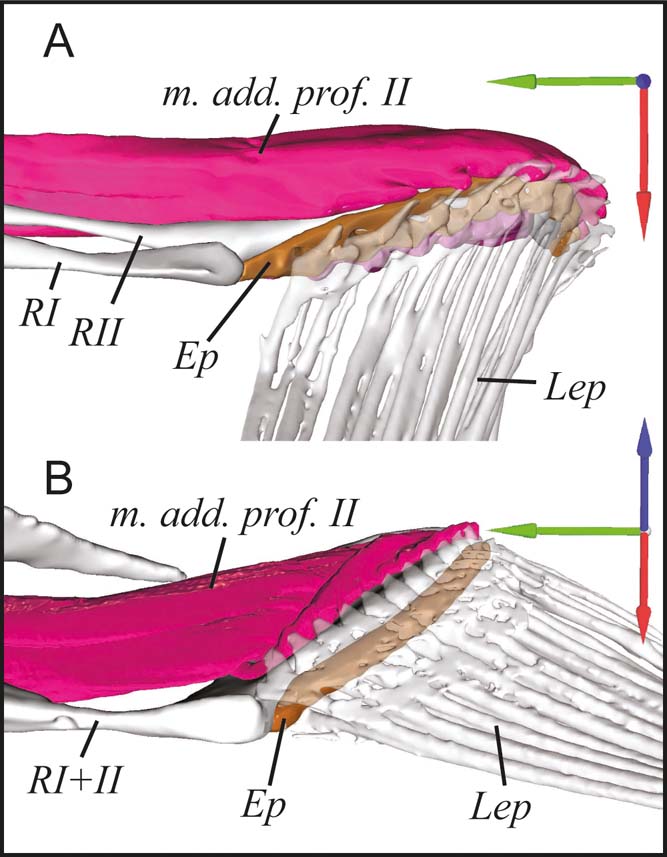
Figure 5 Proximal articulation of the lepidotrichia with the epactinal rod in benthic anglerfish. (A) Antennarius coccineus. (B) Ogcocephalus notatus. Joint coordinate system: blue = dorsal; green = anterior; red = lateral. Abbreviations: Ep = epactinal rod; Lep = lepidotrichia; m. add. prof. II = m. adductor profundus II; RI–RII = radials I and II, or I+II.
The first radial is the most dorsal of the fin bones, articulating proximally with the scapulocoracoid (Fig. 3). In the pelagic species, radial I is very small and has no distal articulation. In benthic species, radial I or I+II is much larger and the distal end articulates with the cartilaginous epactinal rod. Further, in Antennarius, radial I articulates proximally with radial III+IV, and in Ogcocephalus, radial I+II fuses proximally with radial III+IV forming the concave surface of the reverse ball-and-socket glenoid (Fig. 4).
The second radial is only present in pelagic species and Antennarius, lying ventral to radial I (Fig. 3). In Cryptopsaras, radial II is morphologically similar to radials III and IV, articulating proximally with the scapulocoracoid and distally with the lepidotrichia; in Haplophryne, radial II is reduced to a splint between radial I and III and has no apparent articulations; in Antennarius, radial II is the smallest radial and is fused proximally and distally with radial III+IV, acting as a diagonal strut between radial I and III+IV.
Radials III and IV are unremarkable in Cryptopsaras, similar in morphology to radial II, articulating proximally with the scapulocoracoid and distally with the lepidotrichia (Fig. 3a). These three radials are notched distally, with radial II inserting into the notch on radial III, and radial III inserting into radial IV. Radial III in Haplophryne is highly modified into a broad sickle shape, contributing to a large portion of the fin and concentrating most of the distal area for muscle attachment (Fig. 3a). Radial IV is broadly notched distally for the reception of radial III.
In both benthic species, radial III+IV is large, robust, and distally expanded, creating a large area for muscle attachment (Fig. 3b). Proximally, radial III+IV articulates with the scapulocoracoid; in Antennarius radial III+IV also articulates medially with radial I, and in Ogcocephalus it is fused medially with radial I+II (Fig. 4). This medial connection between radials creates a larger surface for movement at the reverse ball-and-socket glenoid joint. Radial III+IV articulates distally with the epactinal rod (Fig. 5).
2.1.3. Lepidotrichia
The lepidotrichia of Cryptopsaras and Haplophryne are thin and wispy with very simple articulations with the radials (Fig. 3a); scan resolution was insufficient to distinguish any proximodistal segmentation. In contrast, the lepidotrichia of Antennarius and Ogcocephalus are considerably longer and more robust (Fig. 3b). In Antennarius, the lepidotrichia are splayed and each lepidotrich articulates proximally with the epactinal rod and has a larger process on the ‘adductor' side for muscle attachment (Fig. 5). Further, the lepidotrichia are hemispherical in cross section and are unsegmented along the proximal third, becoming segmented and tapering towards the tip. The lepidotrichia of Ogcocephalus appear to be tightly bound (i.e., they do not splay) and are unsegmented throughout; however, similar to Antennarius, each lepidotrich articulates proximally with the epactinal rod and has an enlarged process on the ‘adductor' side for muscle attachment (Fig. 5).
2.2. Muscle morphology
Comparison of pectoral fin muscles between pelagic and benthic anglerfish species shows general conservation in the number of homologous muscles (nine) and the position (origin/insertion) of the muscles. The one major difference is that benthic species have an additional (novel) muscle – the m. transversus – which bridges the shoulder joint. A summary of the following muscle descriptions is provided in Table 2. Muscle actions were inferred by inspecting the origin/insertion and line of action around their primary joint. Due to limitations with scanning resolution, muscle fibres leading to lepidotrichia were difficult to fully segment, particularly in the smaller pelagic species. Therefore, the distal ends of some muscles are not rendered, although their insertion points are noted. Considering how small these fibres are relative to the total size and volume of the whole muscles, they will have had minimal impact on the calculated muscle properties.
Table 2 Muscle data: origin, insertion, inferred action, and measured muscle properties. Volumes, fibre lengths, and PCSAs (physiological cross-sectional areas) are presented as raw data and normalised to body mass (bold).
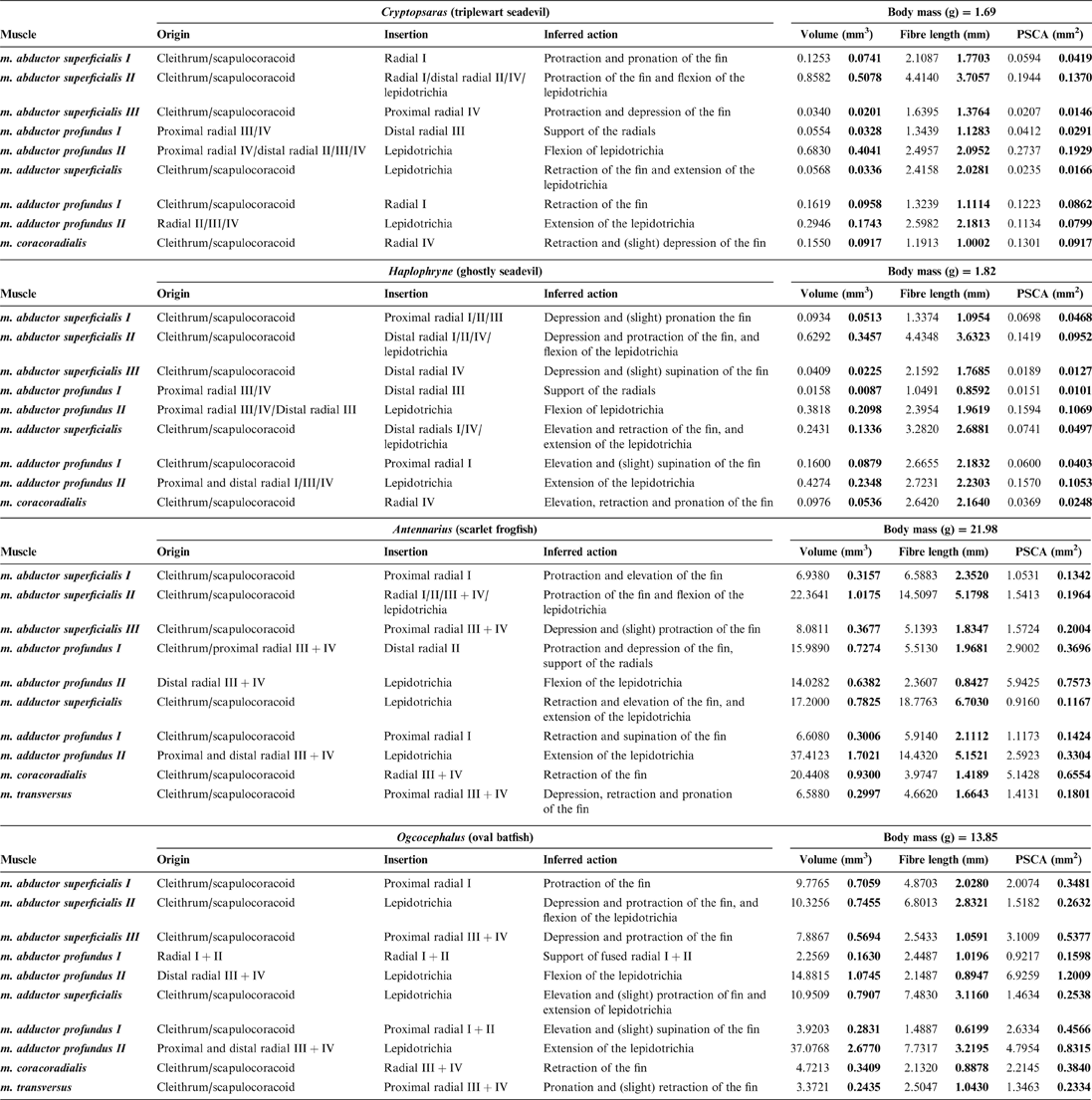
2.2.1. ‘Abductor' muscles
The superficial layer of the ‘abductor' surface of the pectoral fin is made up of the m. abductor superficialis I, II, and III (Figs 6, 7). The m. abductor superficialis I is relatively small. It originates on the cleithrum/scapulocoracoid and inserts proximally onto radial I, II, and III in Haplophryne, but only radial I in all other species (Fig. 3; Table 2). Its primary action in Haplophryne is to protract and pronate the fin; in Cryptopsaras, to depress and slightly pronate the fin; in Antennarius, the muscle inserts more dorsally, and so instead protracts and elevates the fin; and in Ogcocephalus, it functions to protract the fin (Table 2).
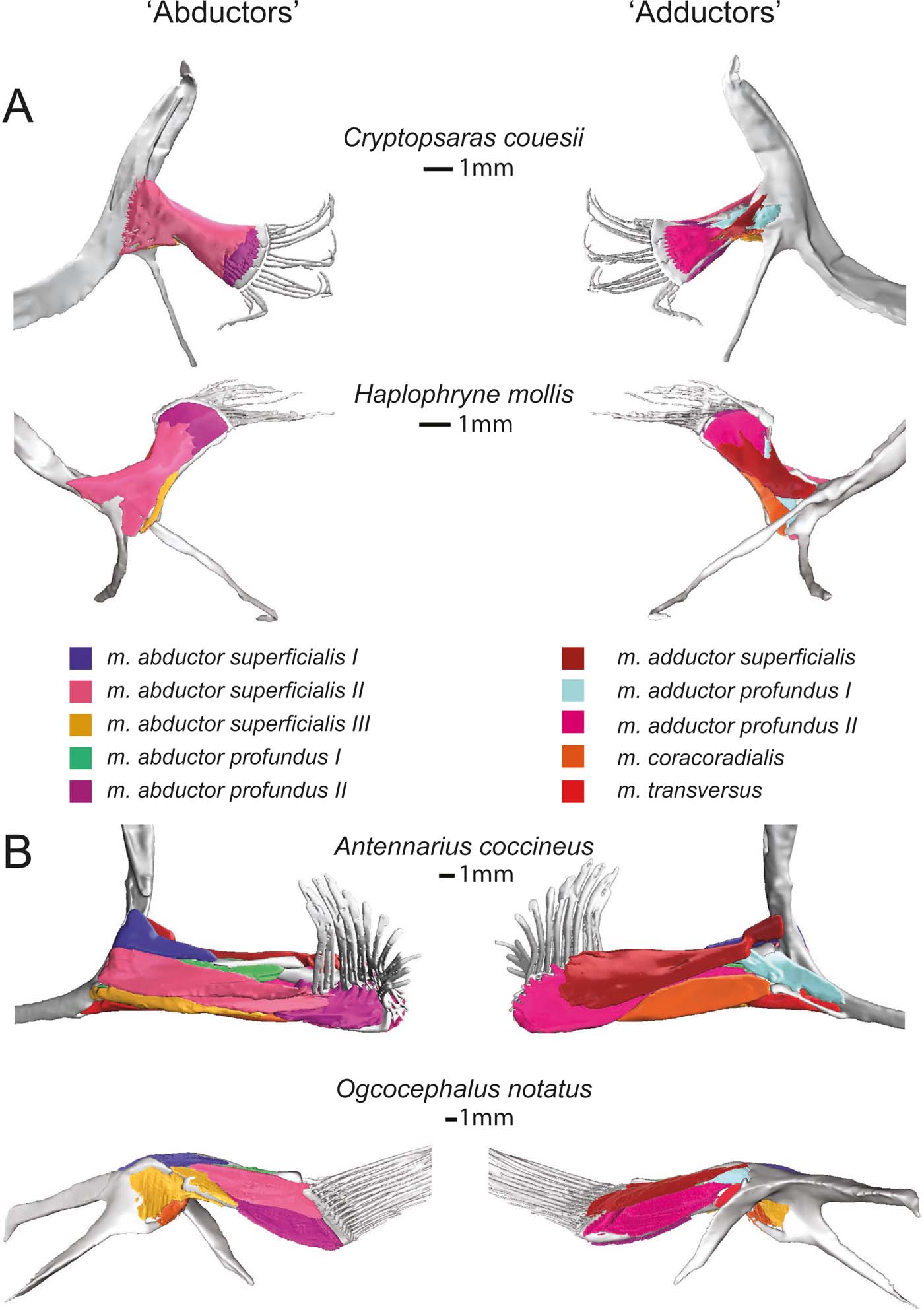
Figure 6 Superficial (and deep) musculature from the ‘abductor' (left) and ‘adductor' (right) surfaces. (A) Pelagic species Cryptopsaras couesii and Haplophryne mollis. (B) Benthic species Antennarius coccineus and Ogcocephalus notatus. Muscles are colour coded for homology.

Figure 7 Deep (and superficial) musculature from the ‘abductor' (left) and ‘adductor' (right) surfaces. (A) Pelagic species Cryptopsaras couesii and Haplophryne mollis. (B) Benthic species Antennarius coccineus and Ogcocephalus notatus. Muscles are colour coded for homology.
The morphology of the m. abductor superficialis II is fairly consistent between pelagic and benthic species (Fig. 6). It is the largest and most superficial muscle of the ‘abductor' musculature, originating on the cleithrum/scapulocoracoid and inserting distally onto all the radials and bases of the lepidotrichia (Fig. 3; Table 2). The primary action of the m. abductor superficialis II is to protract the fin and flex the lepidotrichia (Table 2). However, it also acts to depress and protract the fin in both Haplophryne and Ogcocephalus.
The m. abductor superficialis III (Figs 6, 7) originates on the cleithrum/scapulocoracoid and inserts onto the ventral-most radial: radial IV in pelagic species and radial III+IV in benthic species (Fig. 3; Table 2). The action remains consistent among Cryptopsaras, Antennarius, and Ogcocephalus, where it depresses and protracts the fin. In Haplophryne, it instead acts to depress and supinate the fin (Table 2).
The deep ‘abductor' muscles include the m. abductor profundus I and II (Figs 6, 7). In all species except Antennarius, the m. abductor profundus I is small in size, located between the radials, acting only to support the radials (Figs 3, 7; Table 2). In Cryptopsaras, Monod (Reference Monod1960) identified an insertion on radial II, though we could not confirm this. It is highly reduced in Ogcocephalus, due to the fusion of radials I and II: it exists only as a thin sheet with a thread of muscle passing through a small foramen in the fused radials I+II (Figs 3b, 7b). This muscle is larger in Antennarius, originating on the cleithrum/scapulocoracoid and proximal radial III+IV; in addition to supporting the radials, it also acts to protract and depress the fin (Figs 3b, 7b; Table 2).
In all species, the m. abductor profundus II (Figs 6, 7) inserts on and acts solely to flex the lepidotrichia, though its origination varies among the species (Fig. 3; Table 2). In Cryptopsaras, it originates proximally on radial IV, and more distally on radials II, III, and IV. In Haplophryne, it originates proximally on radials III and IV, and more distally on radial III. In both benthic species, it originates on the distal end of the fused radial III+IV. In Ogcocephalus, due to the extreme rotation of the fin and action about the epactinal rod, the m. abductor profundus II will also act to retract the lepidotrichia.
2.2.2. ‘Adductor' muscles
The only superficial muscle on the ‘adductor' surface of the pectoral fin is the m. adductor superficialis (Fig. 6), the assumed antagonist to the m. abductor superficialis II. In all species, it originates dorsally on the cleithrum/scapulocoracoid and inserts on the proximal bases of the lepidotrichia; it also inserts distally on radials I and IV in Haplophryne (Fig. 3; Table 2). It is very thin and sheet-like in the pelagic forms, which made it difficult to segment. Its action on the fin varies between species, though it does extend the lepidotrichia in all species (Table 2). In Cryptopsaras, it also acts to retract the fin; in Haplophryne and Antennarius, to retract and elevate the fin; and in Ogcocephalus, to elevate and slightly protract the fin.
There are two deep adductor profundus muscles (Figs 6, 7). In all species, the m. adductor profundus I originates on the cleithrum/scapulocoracoid and inserts proximally onto radial I or I+II in Ogcocephalus (Fig. 3; Table 2). In Cryptopsaras, it acts to retract the fin; in Haplophryne, to elevate and slightly protract the fin; in Antennarius, to retract and supinate the fin; and in Ogcocephalus, to elevate and slightly supinate the fin (Table 2). Unlike all other muscles in the fin, the m. adductor profundus I is of similar relative size in all the species studied (Figs 6, 7).
The m. adductor profundus II is the largest muscle on the ‘adductor' surface of the pectoral fin (Fig. 6) and is by far the largest fin muscle by volume in benthic species. It originates both proximally and distally on the radials (Fig. 3; Table 2): II, III, and IV in Cryptopsaras; III in Haplophryne; and fused radials III+IV in both benthic species. It inserts at the base of the lepidotrichia in all species, acting to extend the lepidotrichia (Fig. 3; Table 2).
The m. coracoradialis is the last deep muscle that all species have in common (Figs 6, 7). It originates on the cleithrum/scapulocoracoid and inserts onto radial IV in pelagic species and radial III+IV in benthic species; it generally acts to retract the fin in benthic forms and takes on further actions in pelagic species (Fig. 3; Table 2). In Antennarius, this muscle is relatively very large (Fig. 7).
A final deep muscle, the m. transversus, is only found in Antennarius and Ogcocephalus (Figs 6b, 7b). In both animals, it originates on the cleithrum/scapulocoracoid and inserts on radial III+IV (Fig. 3; Table 2). However, its primary action on the fin differs: it has the combined action of depressing, retracting, and pronating the fin in Antennarius, while in Ogcocephalus it acts to pronate and only slightly retract the fin (Table 2).
2.3. Muscle properties
Overall, the two benthic anglerfishes (compared with the pelagic species) have substantially larger muscle volumes relative to body mass, with the m. adductor profundus II being the largest (Figs 8, 9; Table 2). As mentioned in the previous section, the m. adductor profundus II extends the lepidotrichia (Table 2). Muscle fibre lengths, an indicator of muscle displacement, are generally much longer relative to body mass in Antennarius compared to the other species (Figs 8, 9; Table 2) – particularly the m. abductor superficialis II, m. adductor superficialis, and m. adductor profundus II. These three muscles largely facilitate protraction/retraction at the shoulder and movement of the lepidotrichia (Table 2). The only instances where Antennarius does not have the longest fibres is m. abductor profundus II (flexion of lepidotrichia), where both pelagic species have the longest fibres, and m. coracoradialis, which is longest in Haplophryne. In Haplophryne, the origin and insertion points of the m. coracoradialis indicates that it mobilises the fin along multiple anatomical axes (Table 2). Muscle PCSA, an indicator of force, is much greater in the benthic species compared to the pelagic species (Figs 8, 9; Table 2), with the m. abductor profundus II – which acts to flex the lepidotrichia – being the largest. Within the benthic species, Ogcocephalus has the greatest PCSAs in all instances, except for m. abductor profundus I and m. coracoradialis, which are greatest in Antennarius. These two muscles function to protract/retract the fin (Table 2).
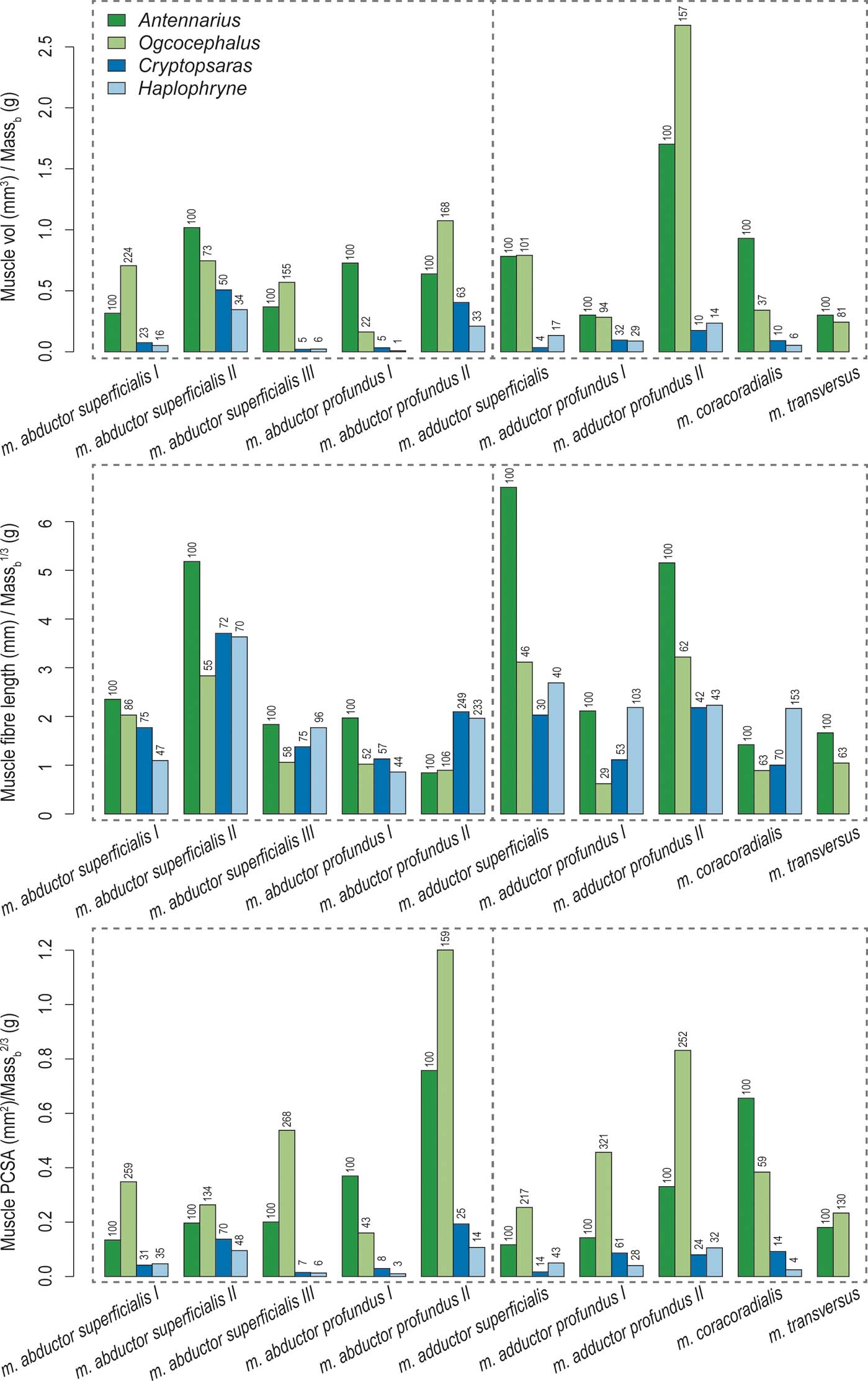
Figure 8 Muscle volume, fibre length, and PCSA of ‘abductor' (left of vertical dotted line) and ‘adductor' (right of vertical dotted line) muscles for each anglerfish species. Values are normalised to body mass using geometric similarity. Numbers above columns indicated relative percent difference from Antennarius.
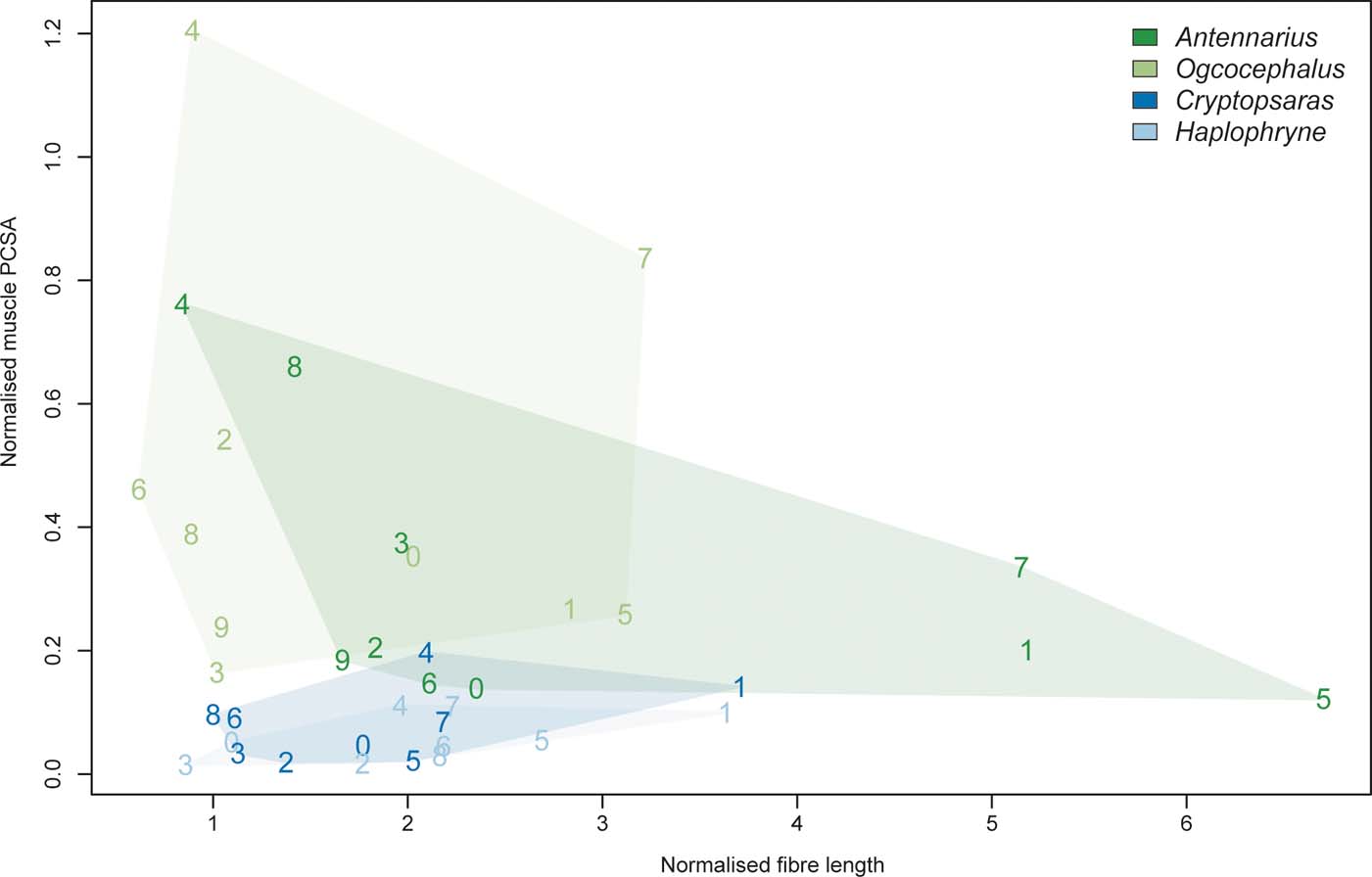
Figure 9 Biplot of normalised muscle PCSA versus normalised fibre length. Muscles are numbered 0–9: 0 = m. abductor superficialis I; 1 = m. abductor superficialis II; 2 = m. abductor superficialis III; 3 = m. abductor profundus I; 4 = m. abductor profundus II; 5 = m. adductor superficialis; 6 = m. adductor profundus I; 7 = m. adductor profundus II; 8 = m. coracoradialis; 9 = m. transversus.
3. Discussion
3.1. Major differences between pelagic and benthic anglerfish fins
Our results clearly show broad musculoskeletal differences between the pectoral fins of pelagic and benthic anglerfish species. In both benthic species, the fin radials are more robust, having undergone fusion and expansion, and they have larger and more differentiated muscles. Further, the proximal ends of the radials articulate or fuse and form a concave surface that results in a reverse ball-and-socket glenoid joint with the scapulocoracoid (Fig. 4). Benthic species have much larger muscles and a greater capacity for force generation relative to body size. These differences may reflect the functional requirements of using the fin to ‘walk' along substrate. Generally, forward propulsion in pelagic fishes is generated by the tail through action of the body muscles, and the function of the pectoral fins is limited to the finer control of speed and direction (Gans et al. Reference Gans, Gaunt and Webb1997). Substrate-driven locomotion depends on the fins interacting with a solid medium to produce motion, which would likely result in higher peak forces due to reduced substrate compliance (Foster et al. Reference Foster, Dhuper and Standen2018). As such, the fins would not only need to be strong enough to resist the larger forces of pushing against a solid, but also flexible and dextrous enough to produce meaningful strides. We also find differences between our two benthic species. As compared to the batfish, the pectoral fin of the frogfish has less bony fusion, smaller PCSAs (or capability to produce force), and longer muscle fibres (or greater muscle displacement). In the following sections we detail how these musculoskeletal differences may help to support divergent fin-assisted locomotion behaviours.
3.2. Osteological adaptations of the pectoral fin for ‘walking'
The number and morphology of fin radials varies considerably across anglerfish. Monod (Reference Monod1960) observed a general pattern in the reduction of radials across the anglerfishes and attempted to reconstruct a sequence of reduction of these bones, with five radials being the ancestral state, reducing down to two through several pathways. While this seems reasonable, modern phylogenetic analyses of anglerfishes are in flux (e.g., Miya et al. Reference Miya, Pietsch, Orr, Arnold, Satoh, Shedlock, Ho, Shimazaki, Yabe and Nishida2010), making it difficult to establish the evolutionary pattern. It is clear, however, that compared to the pelagic anglerfish species examined here, the benthic substrate locomotors have fewer, longer, and more robust radials in their pectoral fins (Fig. 3). Reduced numbers of radials in the frogfish and batfish, to three and two respectively, likely represents a strategy to increase bone stiffness (and area for muscle attachment). Fusing smaller bones into a single larger bone has the effect of increasing mechanical strength and resistance to bending, without necessarily needing to increase the amount of bony material (Currey Reference Currey2013; McHorse et al. Reference McHorse, Biewener and Pierce2017). The reduction of limb bones, either through fusion or loss, is a common theme in animals needing to optimise bone strength. This is often seen in large terrestrial animals experiencing particularly strenuous locomotion, such as horses (e.g., Biewener et al. Reference Biewener, Thomason and Lanyon1988; McHorse et al. Reference McHorse, Biewener and Pierce2017) and hopping marsupials (e.g., Bennett Reference Bennett2000), but this strategy could be useful in many ecological situations that put high stress on bones.
The reverse ball-and-socket joint possessed by frogfish and batfish (and absent in the seadevils) will act to increase both mobility and stability of the glenoid joint (Fig. 4). This type of joint morphology has convergently evolved numerous times in fishes that frequently engage in fin–substrate interaction: epaulette sharks (Goto et al. Reference Goto, Nishida and Nakaya1999), sand skates (Lucifora & Vassallo Reference Lucifora and Vassallo2002), bichirs (Wilhelm et al. Reference Wilhelm, Du, Standen and Larsson2015); and lobe-finned fishes (Edwards Reference Edwards1989; Miyake et al. Reference Miyake, Kumamoto, Iwata, Sato, Okabe, Koie, Kumai, Fujii, Matsuzaki, Nakamura and Yamauchi2016). Of course, the ball-and-socket joint is a major feature of crown tetrapod limbs (especially proximal joints); a feature that enables them to perform a diverse array of locomotor behaviours (Edwards Reference Edwards1989). Interestingly, during the transition from tetrapodomorph fish to early tetrapods, the ball-and-socket shoulder joint seems have been temporarily modified into an anteroposteriorly elongate and dorsoventrally flattened glenoid fossa; this would have resulted in a condyloid-like joint that permitted movements along the primary and secondary axes of the shoulder (anterior/posterior; dorsal/ventral), but greatly restricted rotary motions (Pierce et al. Reference Pierce, Clack and Hutchinson2012, Reference Pierce, Hutchinson and Clack2013). Such a morphological signature may indicate that early tetrapods employed different joint kinematics to modern tetrapods and certain ‘walking' fish, which may have resulted in a more limited locomotor repertoire.
In the frogfish and batfish, the lepidotrichia (or fin rays) are proportionally longer and more robust as compared to the pelagic seadevils (Figs 2, 3). Although our scan resolution made it hard to examine the internal anatomy of the fin rays, an increase in size may also indicate greater cross-sectional area and, in turn, the ability to withstand greater forces generated by contact with substrate. In both benthic species, the fin rays are flexed ventrally compared to the radials creating the appearance of a ‘wrist' – allowing the fin rays to contact the substrate. The ‘wrist-like' flexion/extension is supported by larger muscles that attach to enlarged processes on the proximal ends of the lepidotrichia (Figs 3, 5). Mudskippers, which are arguably the most efficient terrestrial ‘walking' fish, also have a flexible ‘wrist' between their radials and fin rays which they use to propel the body forward (Pace & Gibb Reference Pace2009). The batfish fin rays are exceedingly long, straight, and appear to function as a unit; i.e., there is little inter- or intra-ray movement. Conversely, the distally segmented fin rays of the frogfish take on the appearance of tetrapod ‘fingers', allowing them to grip objects such as corals and rocks (Edwards Reference Edwards1989; Lauder et al. Reference Lauder, Madden, Mittal, Dong and Bozkurttas2006; Taft et al. Reference Wilhelm, Du, Standen and Larsson2008). Regional segmentation is also found in the pectoral fin rays of the longhorn sculpin (Myoxocephalis octodecimspinosus), and may be an important adaptation for substrate-driven locomotion (Taft et al. Reference Wilhelm, Du, Standen and Larsson2008). The differences in fin ray morphology between the frogfish and batfish are in keeping with their ecological niche; frogfish live in closer association with reefs where they frequently grip objects, while batfish occupy more open seabeds with fewer obstacles (Froese & Pauly Reference Froese and Pauly2018).
3.3. Myological adaptations of the pectoral fin for ‘walking'
The biggest myological difference between pelagic and benthic anglerfish pectoral fins is size. Both the frogfish and batfish have comparatively large muscle volumes and PCSAs (Figs 8, 9; Table 2), with clearly defined muscle bellies (Figs 6, 7). The pelagic seadevils have more poorly differentiated muscles which are sheet-like in morphology. Consequently, the muscles of benthic substrate locomotors have a greater capacity to generate force. The relatively long radials of both benthic species also displace the muscle insertions further distally – meaning muscles are not only more forceful but have better leverage for movement. Further, long radials will result in a greater range of movement of the fin for a given angular displacement of the glenoid joint or range of muscle shortening (Lieber Reference Lieber2002; Biewener & Daley Reference Biewener and Daley2007). Out of the nine homologous muscles (Monod Reference Monod1960), the m. adductor profundus II is the largest muscle by volume in the benthic species, while the m. abductor profundus II is the largest by PCSA; these antagonistic muscles act to extend and flex the fin rays or ‘wrist', respectively. Presumably, this flexion/extension of the ‘wrist' assists benthic species to ‘walk' along the substrate, and frogfish to grip and release objects – similar to a tetrapod hand. Finally, frogfish and batfish have an additional muscle on the ‘adductor' surface of the fin – the m. transversus. This muscle crosses the shoulder joint but its insertion is very proximally located, indicating poor leverage. Thus, the m. transversus may contribute more to shoulder joint stabilisation – perhaps for station-holding – rather than powerful ‘walking' movements.
Comparing the benthic species, we see further modifications for specific locomotor ecology. The frogfish has the proportionally longest muscle fibres, with the three longest muscles being: m. abductor superficialis II, m. adductor superficialis, and m. adductor profundus II (Figs 8, 9; Table 2). These muscles primarily act to protract/retract the fin and flex/extend the fin rays. Long muscle fibres have a longer active working range (or range of motion), meaning they can produce greater force for a given length than shorter fibres (Lieber Reference Lieber2002; Biewener & Daley Reference Biewener and Daley2007). As frogfish tend to use extensive fin mobility to ‘walk' around their complex reef environment (Pietsch & Grobecker Reference Pietsch and Grobecker1987), long fibres would allow them to maintain muscle force at various stages of a stride and while gripping and releasing objects. In terms of force generation capacity, the batfish pectoral fins are the most effective, with the proportionally largest overall PCSAs (Figs 8, 9; Table 2). The two muscles with the largest PCSAs are the m. abductor profundus II and the m. adductor profundus II, an antagonistic pair of muscles that flex and extend the fin rays, respectively. Batfish tend to ‘walk' on more even substrate in open seabeds (Froese & Pauly Reference Froese and Pauly2018), and online videos indicate they use far less proximal fin mobility than frogfish, with the majority of forward momentum coming from flexion/extension of the ‘wrist' (articulation between radials and fin rays). Further, they can use their fins in combination with their tail to swim in short bouts. Thus, our muscle data demonstrate a classic trade-off between range of movement and force generation in ecologically diverse walking anglerfish: the frogfish pectoral fin musculature supports a large range of fin movements to navigate complex reefs, while the batfish pectoral fin musculature supports more powerful (but more restricted) movements on flat seabeds.
3.4. Implications (speculations) for the evolution of limbs
Many of the adaptations described here are also found in tetrapods, as well as other fishes that use their fins in limb-like ways. For instance, a (reverse) ball-and-socket glenoid joint has repeatedly evolved in animals as disparate as sharks/rays (Goto et al. Reference Goto, Nishida and Nakaya1999; Lucifora & Vassallo Reference Lucifora and Vassallo2002), frogfishes (Edwards Reference Edwards1989; this study), lungfishes (Edwards Reference Edwards1989), and tetrapods, which may indicate proximal joint mobility/stability is important for fin/limb–substrate interactions. The presence of more distally located joints also appears to be a trend; for instance, frogfish, batfish, and mudskippers (Harris Reference Harris1960; Pace & Gibb Reference Pace and Gibb2009) have ‘wrist-like' joints between their radials and fin rays that facilitate fin ray movements, and tetrapods have both an elbow and a wrist. These additional joints have the effect of increasing the degrees of freedom, which, in turn, enhances fin/limb mobility. Frogfish and sculpins (Taft et al. Reference Taft, Lauder and Madden2008) also show regional segmentation of their fin rays, which invokes similarity to multi-jointed tetrapod fingers, a morphological feature that provides dexterity. Another commonality found between ‘walking' anglerfish and tetrapods is the development of discrete muscle bellies rather than muscle sheets – allowing for finer control of fin/limb movements. Molnar et al. (Reference Molnar, Diogo, Hutchinson and Pierce2017) recently detailed the evolution of tetrapod forelimb muscles from the dorsal and ventral muscle sheets of sarcopterygian fish. The study found that muscles began to differentiate in tetrapodomorph fish but that the full complement of tetrapod forelimb muscles coincides with the evolution of limbs (and digits). Considering the anatomical similarities between walking fishes and tetrapods, it is not unreasonable to hypothesise that any type of fin–substrate interaction may provide selective pressure to turn a fin into a limb. Perhaps the degree of limb-like adaptations depends on the type (and combination) of fin–substrate interactions (station-holding, walking, gripping) and the complexity of the environment being traversed.
4. Conclusion
Comparing pectoral fin musculoskeletal anatomy of pelagic swimming anglerfish with benthic substrate locomotors has highlighted a number of potential adaptations that may enable fin-assisted ‘walking'. (1) Radials and fin rays are more robust, likely resulting in stronger bones better capable of resisting substrate reaction forces and the forces imposed by muscles. (2) Radials and fin rays are elongated, providing greater overall fin movement for a given joint angular displacement, effectively leading to increased stride length. (3) The glenoid fossa forms a reverse ball-and-socket joint, a shape expected to impart greater range of proximal fin mobility and joint stability. (4) Fin rays articulate with the radials at a mobile ‘wrist' joint, increasing fin degrees of freedom and supporting enhanced distal fin manoeuvrability. (5) Muscles are much larger and form discrete muscle bellies (rather than sheets), leading to a greater capacity to generate force during ‘walking' (and other associated) behaviours. Further, through our comparative lens we were able to identify specific musculoskeletal adaptations in frogfish versus batfish that appear to be associated with their distinct benthic locomotor ecologies. Frogfish pectoral fin muscles have longer fibres, which increase their working range; this supports greater fin mobility, allowing them to navigate through complex, 3D reef systems. Conversely, batfish have shorter muscles with large PCSAs, resulting in more powerful but less mobile fins; this is in keeping with their more open, 2D seabed habitat.
5. Acknowledgements
This paper was inspired by the research career of Professor Jennifer A. Clack and her contributions to our understanding of the origin and evolution of tetrapods. I (Stephanie Pierce) feel very honoured to have been mentored by Jenny during my early career; she fostered my enthusiasm for animal evolution and the fossil record, imparted her experience and unbounding knowledge, and supported me during my academic development. Now I get to share my knowledge with my own students, including my graduate student (and co-author of this paper) Blake Dickson. In support of this research, we would like to thank the MCZ Ichthyology Collection for access to specimens, including George Lauder, Karsten Hartel, and Andrew Williston. Additional thanks go to the Pierce Lab for informative conversations on the manuscript, as well as critical feedback from Michael Coates and an anonymous reviewer. Funding for this research was provided by Harvard University and published by a grant from the Wetmore Colles fund.