The relationship between dietary fibre and the gut microbiota is complex, and there is still much that we do not understand. The physiological impact of this relationship can vary depending on many different factors including the type of fibre consumed, background diet, gut microbiota composition as well as variations in how these affect digestive function and sensitivity along the gastrointestinal tract of individuals.
Dietary fibre is a diverse group of non-digestible carbohydrates that differ in structure, physicochemical characteristics and physiological effects. Similarly, the gut microbiota is a highly complex, varied and dynamic ecosystem that differs considerably between individuals and in response to extrinsic and intrinsic factors. The composition of the microbiota is thought to be mostly stable in adulthood (60 % over 5 years(Reference Mehta, Abu-Ali and Drew1,Reference Faith, Guruge and Charbonneau2) ), but there may be more changes in metabolic activity than seen in microbiome composition due to functional redundancy and the response to dietary and other perturbations may be difficult to predict for an individual. There is much interaction between bacterial species and groups; some collaborating in the metabolism of carbohydrates and other molecules through cross feeding(Reference Tomlin, Read and Edwards3,Reference Milani, Lugli and Duranti4) . Competition between species can occur by the production of bacteriocins, inhibitory metabolites and low pH as well as by occupying and blocking binding sites on foods particles and mucosa. A varied diet may be more important for determining diversity and stability of the microbiota than supplementation with single foods(Reference Johnson, Vangay and Al-Ghalith5).
Diet in early life, both breastfeeding and weaning, clearly plays an important role in gut colonisation in the human infant(Reference Oluwagbemigun, O'Donovan and Berding6). Less is understood about later determinants of gut microbiome composition, but diet is likely to be one of the biggest influences. Several dietary components could influence individual bacteria species directly or via alterations in gut transit and digestive functions but the main influence is likely to be through dietary fibre. Although dietary fibre and the gut microbiota have their own well established physiological effects, it is the interaction between these two that has drawn growing interest(Reference Cronin, Joyce and O'Toole7–Reference Myhrstad, Tunsjø and Charnock9).
Dietary fibres and their properties
The term dietary fibre encompasses a wide range of carbohydrates and associated molecules in naturally occurring plant structures or as extracted or synthesised molecules. Dietary fibre was originally defined as carbohydrate polymers within plant cell wall structures that escape digestion and absorption in the small intestine(Reference Trowell and Burkitt10). However, the definition was later expanded to include non-digestible polysaccharides that are not situated within plant cell walls, including storage polysaccharides (galactomannans), exudates (gum arabic) and mucilages (ispaghula). There is also a significant proportion of starch in the diet (approximately 10 %) which is resistant to digestion (resistant starch) by being entrapped in the seed, plant cellular structure or otherwise structurally unavailable to amylase in the gut(Reference Englyst and Hudson11,Reference Gallant, Bouchet and Buléon12) . In addition, there are smaller non-digestible carbohydrates in food such as oligosaccharides including those from inulin. Further non-digestible carbohydrates can be synthesised chemically or modified from existing carbohydrates including starch(Reference Laurentin and Edwards13). There are also non-carbohydrate molecules such as lignin and non-extractable polyphenols that are hard to separate from the fibre and which moreover influence and are metabolised by the gut microbiota. The international CODEX Alimentarius Commission debated a new definition for dietary fibre for over 15 years. The final agreed definition (2009)(Reference Jones14) is detailed below and was used as the basis of the European Food Safety Authority definition of dietary fibre in their scientific opinion on dietary reference values for carbohydrates and dietary fibre(15) and in the UK Scientific Advisory Committee on Nutrition 2015 report on carbohydrates and health(16):
Non-starch polysaccharides, all resistant starches, all non-digestible oligosaccharides with three or more monomeric units and other non-digestible, but quantitatively minor components that are associated with dietary fibre polysaccharides, especially lignin.
Additional European Union regulation outlines three categories to which substances classified as dietary fibre must belong(17):
• edible carbohydrate polymers naturally occurring in the food as consumed,
• edible carbohydrate polymers which have been obtained from food raw material by physical, enzymatic or chemical means and which have a beneficial physiological effect demonstrated by generally accepted scientific evidence and
• edible synthetic carbohydrate polymers which have a beneficial physiological effect demonstrated by generally accepted scientific evidence.
Recommended fibre intakes and why it is difficult to meet them
Current dietary guidelines recommend adults consume at least 30 g fibre daily. Data from the National Diet and Nutrition Survey shows that average intake of dietary fibre in UK adults aged 19–64 is 19⋅7 g with only 9 % meeting the recommendation(Reference Roberts, Steer and Maplethorpe18). Fibre intake in childhood is also low although current recommendations are extrapolated from adults rather than based on robust evidence(Reference Edwards, Xie and Garcia19). Nevertheless, early dietary exposure in infancy, e.g. human milk and diet diversity including complex carbohydrates, promotes a more diverse microbiome from early years(Reference Ku, Kim and Lee20). Even when the 30 g daily recommendation is presented in terms of actual food intake, such as by the British Nutrition Foundation's diet plan(21), it is clear that the nations' current average dietary pattern is far from ideal in terms of fibre intake. Currently, the main sources of fibre in the diets of UK adults are cereals and cereal products (38 %), vegetables and potatoes (30 %), meat and meat products (12 %) and fruit (8 %)(Reference Roberts, Steer and Maplethorpe18). Inclusion of fibres in processed foods or fibre supplements may aid individuals in meeting this target but these should not be relied on as primary fibre sources. Naturally, fibre-rich foods have lower energy density and are often sources of vitamins, minerals, as well as other potential microbial substrates such as polyphenols. Ideally, fibre intake should be sourced from a range of different foods throughout the day which will provide a variety of fuel and nutrients for the microbiota and promote bacterial diversity(Reference Röytiö, Mokkala and Vahlberg22,Reference Pu, Li and Du23) .
However, it is important to understand the barriers to increased fibre consumption, these are complex and often associated with social determinants of health but also with understanding and beliefs that can be modified. These include limited knowledge of the impact of fibre on health and its role in promoting a healthy gut microbiome, resistance to behaviour change, hedonic preferences, negative perceptions of insoluble fibre-rich foods and perceived cost and food preparation barriers(Reference Hooper, Spiro and Stanner24). Some fibre-rich foods may also initially increase gut symptoms related to microbial gas production discouraging fibre intake. Moreover, there are many smaller non-digestible carbohydrates in food, including inulin-type oligosaccharides and poorly absorbed sugars and polyols, which increase symptoms in irritable bowel syndrome(Reference Halmos, Power and Shepherd25). These fermentable oligosaccharides, disaccharides, monosaccharides and polyols can have osmotic effects in the intestine leading to increased fluid retention and are also rapidly fermented by the intestinal bacteria producing gas causing distension, flatulence and discomfort.
Fibre consumption and interactions with other food components
As with other dietary components and nutrients, fibres are not generally consumed in isolation. Fibres are usually present in our diet as mixtures and complexes; it is rare to eat isolated types of fibre unless as a supplement. When consumed in whole foods or meals, fibres are generally present as integrated complex matrices of non-digestible components. Healthful dietary components such as polyphenols, vitamins and minerals such as calcium are also associated with fibrous foods. The fibre matrix can trap these components, preventing absorption in the upper intestinal tract. In the colon, bacterial degradation of fibre can release these trapped molecules allowing absorption into the circulation before or after further metabolism by the microbiota. It is difficult to separate the impact of dietary fibre and non-extractable polyphenols (those integrated into the fibre structure and not released in the small intestine) on the gut microbiome and some of the related potential health benefits suggested for dietary fibre(Reference Gutiérrez-Díaz, Salazar and Pérez-Jiménez26). Moreover, there are clear interactions between polyphenols and fibre which affect the production of bioactive phenolic catabolites by the gut microbiota(Reference Edwards, Havlik and Cong27). Inhibition of phenolic acid production by incubations of human faecal bacteria was greatest for raftiline and pectin in comparison with ispaghula(Reference Mansoorian, Combet and Alkhaldy28). More fermentable fibres such as inulin and resistant maltodextrin had the greatest impact on bacterial catabolism of rutin increasing the production of bioactive phenolic acids e.g. 3,4-dihydroxyphenylacetic acid in vitro (Reference Havlik, Marinello and Gardyne29). Individual polyphenols may have a key role in determining composition of the microbiota as some have potential anti-bacterial properties(Reference Olszewska, Gędas and Simões30) but others may act as probiotics(Reference Tzounis, Rodriguez-Mateos and Vulevic31,Reference Bialonska, Kasimsetty and Schrader32) . Little effect of polyphenols on SCFA production from fibre in vitro has been reported(Reference Mansoorian, Combet and Alkhaldy28,Reference Havlik, Marinello and Gardyne29) .
It is becoming more common for fibres to be present in our diet as additional ingredients in processed food products(Reference Bingley33). This can serve several purposes; to replace fat, reduce sugar and provide texture, emulsification or stability. The amount may be very low as a stabiliser (e.g. guar gum in ice cream) or more significant as with nutrient replacers. The increasing prevalence of diet-related health conditions such as obesity and type 2 diabetes, coupled with increasingly poor diets globally, has necessitated greater focus on reformulation strategies such as these. Moreover, including higher levels of fibre in foods, such as added inulin now being seen in some breakfast cereal brands, may provide added health impact and in future could also be part of the functional food market when sufficient evidence for those products is available.
Not all fibres are the same
Physicochemical structure
There is an extensive variety of different fibres in our diets. These fibres differ in physicochemical characteristics, food matrix, source (natural or synthetic), purity and the dose we are likely to consume. Dietary fibres include non-digestible oligosaccharides (e.g. fructo-oligosaccharides, FOS, and galacto-oligosaccharides) which may be natural or synthetically produced, long chain fructans (e.g. inulin), gums and heteropolymers (e.g. pectin), non-starch polysaccharides (e.g. cellulose), hemicellulose, β-glucans and resistant starch. Traditionally, fibres are classified based on solubility; soluble fibres include pectin, β-glucan and oat fibre, and insoluble fibres include cellulose, wheat bran and resistant starch. Solubility does not necessarily determine how a fibre will behave in the gut; soluble fibres do not all share the same physicochemical properties and similarly with insoluble fibres. Broadly speaking, when soluble fibres such as pectin and β-glucan mix with water, they become viscous (to varying degrees depending on the fibre and dose). This viscosity largely determines the health effects of soluble fibres, such as blood glucose and cholesterol level moderation by reducing the rate of absorption from the small intestine. However, SCFA produced in the colon from the fermentation of dietary fibre can also impact many aspects of lipid and glucose metabolism through interaction with SCFA-activated receptors FFAR2 and 3 in the gut, pancreas and adipose tissues(Reference Bolognini, Dedeo and Milligan34) as well influencing liver metabolism(Reference Morrison and Preston35). Insoluble fibres can also hold water but not as much as viscous soluble fibres such as ispaghula (approximately 8⋅6 g water/g fibre) and β-glucan (approximately 4⋅6 g/g)(Reference McBurney36). This can be beneficial in normalising stool form and preventing constipation if the fibre is not totally fermented in the colon. Ispaghula is a great stool bulker whereas pectin and guar gum are not as they are more readily fermented in the proximal colon and their water-holding capacity is lost.(Reference Edwards and Eastwood37). However, wheat bran is less well fermented so its presence in the colon can stimulate propulsion and increase stool output despite a relatively low water-holding capacity.
Natural plant fibre-rich foods v. processed foods
Fibre-rich foods such as cereals, legumes, pulses, fruit and vegetables generally contain a mixture of soluble and insoluble fibres in different ratios. Foods with added fibre could have one or many different types of soluble or insoluble fibre added for functional, structural or sensory reasons. The structural food matrix will determine the impact fibre has on the gut and how accessible it is to the gut microbiota for fermentation. Plant food structure may differ from processed food structure in this sense. Fibres in plant foods may be tightly integrated in the structural matrix of the plant cell wall, bound to minerals, polyphenols or chemically combined to other components limiting exposure to the intestinal lumen and the gut microbiota(Reference Williams, Grant and Gidley38). Conversely, in some processed foods, fibres are more likely to be dispersed throughout the food structure, making it easier for bacteria to access and degrade them. Fibres dispersed throughout foods will be more exposed to the contents of the intestinal lumen. This could mean they have an increased impact on other dietary components present in the gut and greater potential to exert osmotic activity and influence motility. Low-molecular weight oligosaccharides and fermentable oligosaccharides, disaccharides, monosaccharides and polyols, often used in food products but also found in fruits, legumes, wheat and onions, will have a greater osmotic effect on the gut than high-molecular weight fibres(Reference Grabitske and Slavin39). This can lead to increased water retention in the intestine, which may induce symptoms in individuals, such as those with irritable bowel syndrome who are more sensitive to increased lumenal pressure. The accessibility of fibre in a food will also determine whether any physicochemical interactions between different fibres can take place.
Fibre is increasingly present in processed foods with reduced sugar/fat foods and plant-based meat alternatives where fibre is used to provide texture and simulate the sensory or functional properties of the reduced or replaced ingredient. The use of fibres with prebiotic properties, such as inulin, FOS or galacto-oligosaccharides, in food products or supplements is also becoming more popular as evidence supporting the health benefits of the interaction between these fibres and the gut microbiota grows. The amount of added fibre can be relatively high, within the context of average daily consumption, when used for their prebiotic properties(Reference Stephen, Champ and Cloran40). Average daily consumption of inulin and FOS is estimated to be 3–11 g in Europe(Reference Carabin and Flamm41) and 1–4 g in the United States(Reference Moshfegh, Friday and Goldman42). When inulin and/or FOS are used in the usual concentration in foods and dietary supplements this could equate to the consumption of an additional 5–20 g daily(Reference Stephen, Champ and Cloran40).
Types of fibre and their interactions may be more important that total amount
Both the overall dose and types of fibre consumed are important when considering the impact on gut function. The exact weight of fibre may be less important than the type of fibre eaten when considering individual health effects. For example, β-glucan reduces plasma lipids(43) whereas wheat bran may have much less effect. Similarly, β-glucan is readily fermented by the microbiota whereas wheat bran may be only partially fermented. You cannot equate these effects to the total number of g of fibre. For example, high-molecular weight barley β-glucan was more viscous per g than low-molecular weight barley β-glucan and had more effect on gastric emptying and postprandial glycaemia for the same number of g eaten(Reference Thondre, Shafat and Clegg44). The fibre composition and content of natural foods may vary depending on species, the part of the plant consumed and stage of maturity. Although there is a degree of variation, certain types of foods will generally be good sources of fibres; for example, high levels of the insoluble fibre cellulose, β-glucans and pectin are generally found in cereals and grains, oats, citrus fruits and apples respectively. The dose of fibre in processed foods may be far more variable, however(Reference Stephen, Champ and Cloran40). The typical level of inulin and oligofructose in foods can range from 2 to 30 % and 2 to 50 % weight/weight, respectively(Reference Franck45). The relative contribution of different fibres, from whole or purified sources, to the overall fibre dose consumed will determine the predominant impacts on gut function and fermentative activity of the colonic bacteria.
It is also important to consider that in the large intestine fibres may interact with each other. Thus, fibre combinations in one meal may affect the microbiota differently than each fibre eaten alone(Reference Khan and Edwards46). Moreover, the fibre present in the colon at any one time may be an accumulation of fibre consumed over days from different meals depending on the transit time of the individual. In addition, the movement of viscous substances in the small intestine will generally be slower than non-viscous ones(Reference Lentle and de Loubens47) which can influence the delivery of entrapped nutrients and associated molecules to the colon(Reference Lentle and de Loubens47). Inter-meal mixing of fibres with different viscosities can alter transit times of these substances and influence the accessibility of bacteria for fermentation. The length of time a fibre remains in the colon will depend on many factors including the fermentability and physicochemical characteristics of the fibre itself and that of other fibres, and the presence of other dietary components including water in the colon. The individual's colonic bacterial composition is a major determinant as well as their colonic motility and sensitivity to distension and lumenal stimulants. Less fermentable fibres such as wheat bran may stimulate colonic motility and move the fermentation of other fibres further around the colon. They can also provide more binding sites and surfaces for bacteria so biomass could be increased.
The colonic environment is influenced by the presence and fermentability of fibres and other dietary components that reach it. If a highly fermentable fibre/oligosaccharide which transits quickly through the small intestine due to large osmotic load enters the colon, this changes the environment for the fermentation of other fibres. Dominant bacterial fermenters may switch their activity to focus on the highly fermentable fibre and, over time, dominant fibre sources. Fermentative activity influences colonic pH, which subsequently impacts bacterial growth and metabolism. Some bacteria, such as Bacteroides, can survive over a range of pH values whereas others, including Streptococcus, are inhibited by acidic conditions. A decrease in pH can support the growth of beneficial bacteria such as the butyrate producing Firmicutes (Roseburia)(Reference Ilhan, Marcus and Kang48).
Impact of fibre on gut microbiota, fermentation and gut function
Dietary fibre is important for the gut microbiota and gut function in several different ways. As the primary fuel source for bacterial fermentation, fibre promotes the growth of bacterial populations in the gut and stimulates production of beneficial metabolites such as SCFA and bioactive phenolic acids. Fibre is also a source of molecular structures and provides a solid surface for bacteria to bind on to. This enhances the ability of bacteria to remain in the gut and may be important in the production of biofilms. Dietary fibre also functions as a modulator of gut motility. Viscous fibre, by slowing down mixing of the contents of the small intestine and reducing the absorption of a variety of molecules including glucose, fats, polyphenols, calcium and magnesium, may bring more material into the large intestine than is contained in the fibre itself. Poorly fermented fibres can speed up motility, which in turn may reduce time available for fermentation and the absorption of microbial products. The presence of fibres may also influence the thickness of the gut mucosal layer, the efficiency of lumenal mixing and the strength of the smooth muscle(Reference Chen, Chen and Tian49,Reference Schroeder, Birchenough and Ståhlman50) (Fig. 1).

Fig. 1. Impacts of fibre on the gut microbiota and gut function. GI, gastrointestinal; SI, small intestine; SCFA, short-chain fatty acid; WHC, water-holding capacity. Most fermentation takes place in the proximal colon where the liquid content of the colon is higher. The proposed mechanism by which SCFA beneficially impact gut mucosal thickness and integrity is through inducing mucosal healing and suppressing inflammation. When fibre in the diet is lacking, bacteria degrade the colonic mucus layer. When the diet is rich in fibre, the proportion of mucus degrading bacteria decreases, and the thickness of the colonic mucus layer is re-established. The presence of unfermented remnants and fibres with a large particle size, such as wheat bran will stimulate colonic motility throughout the colon.
Fibre selectivity and the gut microbiota
Individual fibres, if eaten in sufficient amounts may select for certain bacterial species, mainly by acting as an energy source for that species. Examples of studies in which the impact of individual fibres or fibre-rich sources on the composition of the human gut microbiota was studied are presented in Table 1. This has mostly been evidenced for the effects of prebiotics on the microbiota(Reference Cantu-Jungles and Hamaker51). Prebiotics are essentially non-digestible food ingredients that can confer beneficial health effects on the consumer by selectively stimulating the growth and/or activity of certain colonic bacteria, in most cases increasing the levels of bifidobacteria or lactobacilli(Reference Gibson, Hutkins and Sanders52) (Fig. 2). However, dominance of a single-fibre source could also reduce bacterial diversity. Other molecules metabolised by the bacteria, including polyphenols, and phenolic acids can also act to increase or inhibit individual bacterial species(Reference Singh, Singh and Bijalwan53,Reference Vanegas, Meydani and Barnett54) . Having a diversity of fibres in the diet will promote a richly diverse, potentially more stable bacterial population(Reference Vlassopoulos, Lean and Combet55–Reference Pashikanti, de Alba and Boissonneault59) (Fig. 3). This is crucial in maintaining a healthy colonic mucus layer which plays important roles in pathogen defence, protection of the intestinal cells against mechanical and chemical damage and maintaining intestinal homoeostasis(Reference Paone and Cani60). When the diet is lacking in fibre, the proportion of mucus-degrading bacteria increases reducing the thickness of the mucus layer which compromises its functionality. If the diet is rich in fibre, the quality of the gut microbiota improves (increased diversity, stability and reduced mucus degrading bacteria), and the mucus layer is re-established.

Fig. 2. Fibre selectivity and the gut microbiota. To be classified as a prebiotic a fibre must be shown to be selectively utilised by host microorganisms conferring a health benefit. The most extensively studied fibres classed as prebiotics are inulin, fructo-oligosaccharides (FOS) and galacto-oligosaccharides (GOS). Natural sources of these fibres include chicory root, Jerusalem artichoke, wheat, onions, garlic and beans. GOS also occur naturally in human milk.
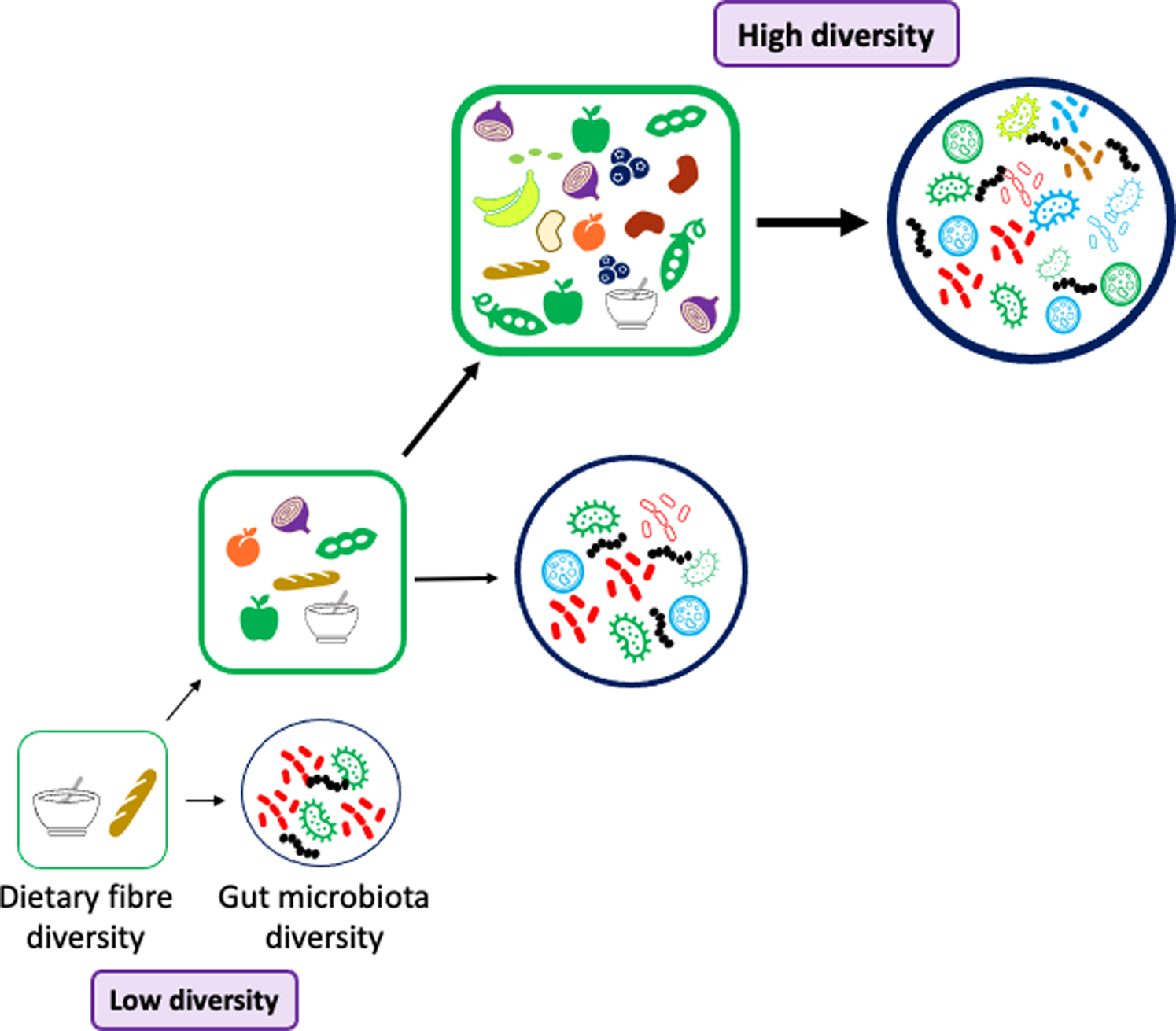
Fig. 3. Dietary fibre and gut microbiota diversity. To promote a diverse and stable gut microbiota population the diet should be rich in a diverse selection of fibres. The foods we consume should contain a range of fibres with different physicochemical properties: solubility, viscosity, water-holding capacity, binding abilities, fermentability, monosaccharide composition, molecular weight and chain length. Many fibrous foods also contain bioactive molecules such as polyphenols which will promote bacterial diversity in the gut e.g. berries, cocoa powder and dark chocolate, beans and fruits including blackcurrants, plums and apples.
Table 1. Examples of human studies investigating the impact of isolated fibres and cereal fibres on the gut microbiota
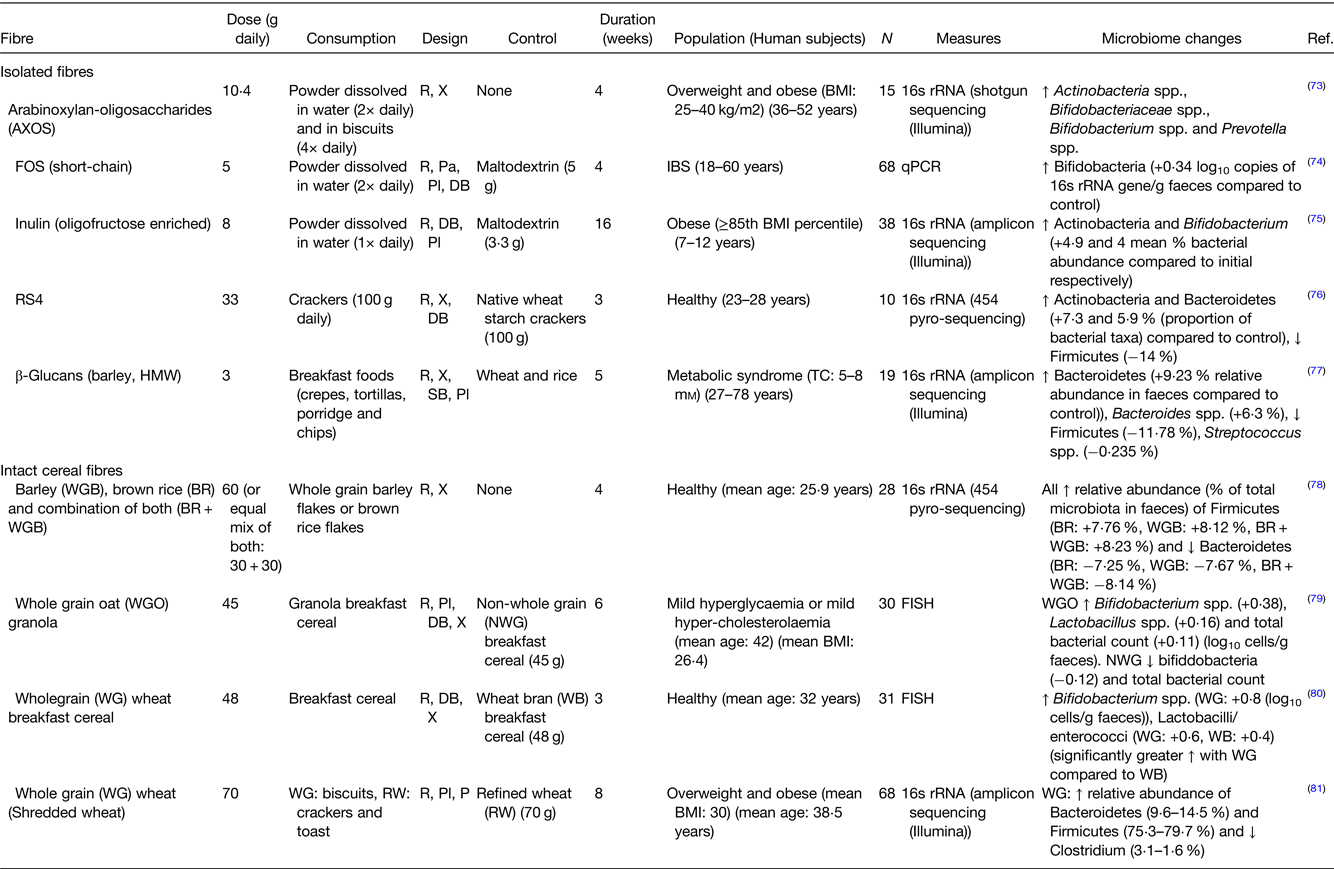
AXOS, arabinoxylan-oligosaccharides; FOS, fructo-oligosaccharides; RS4, resistant starch type 4; HMW, high-molecular weight; IBS, irritable bowel syndrome; R, randomised; X, crossover; Pa, parallel; Pl, placebo; DB, double blind.
Microbial fermentation of different types of fibre
The profile of fermentation differs depending on the type of fibre; patterns of SCFA production, the speed and extent of fibre fermentation and the site of fermentation may all vary. Oligosaccharides and lactulose are rapidly fermented whereas cellulose is hardly fermented at all. Generally, soluble fibres are more rapidly fermented than insoluble fibres, however, this does not hold true for the soluble fibre gellan which is poorly fermented. Much of the variation will be due to the microbiota composition(Reference Feng, Mikkelsen and Hoedt61). The fermentability of a fibre determines the site of fermentation which is important in terms of location of metabolite production and subsequent physiological effects. The more fermentable a fibre is, the more proximally in the colon it will be fermented. Slowly fermented fibres may stimulate colonic motility and promote fermentation in the distal colon. Slowly fermented fibres may also push material through the colon and the fermentation of rapidly fermented carbohydrates may be delayed as a result(Reference Morita, Kasaoka and Hase62). This may spread the production site of SCFA throughout the length of the colon, potentially increasing their benefits.
Products of fermentation
The production of SCFA can be influenced by the dietary fibre source. It is clear that the proportion of acetic, propionic and butyric acids can be characteristic of different fibres. The molar proportion of acetate is increased by pectin fermentation, the proportion of propionate is increased by β-glucan, pyrodextrins and laminarins amongst others(Reference Harris, Morrison and Edwards63) whereas resistant starch and FOS increase the molar proportion of butyrate. The reasons for this selectivity are not clear. In the case of glucose-based polysaccharides, the solubility of the fibre may be key and also the presence of β bonds between the constituent sugars in polysaccharides such as β-glucans, and pyrodextrins in contrast to the α bonds between the glucose units in starch(Reference Laurentin and Edwards13). To try and understand the factors influencing propionate production a series of in vitro fermentation studies were carried out with all possible α and β bonds (bar one) with glucose disaccharides(Reference Harris, Edwards and Morrison64). However, there was no clear pattern of the impact of bond type and the relative production of propionate. In a recent systematic scoping review(Reference Harris, Morrison and Edwards63), the impact of the fermentation of individual carbohydrates on the pattern of SCFA production in vitro was examined. Secondary analysis was used to convert the data in the studies found to normalise the unit of production to either mmol SCFA/g carbohydrate daily or per hour as different studies use a variety of fibre doses, culture volumes and fermentation times which make it difficult to compare their results directly. Twenty-nine substrates were considered. Although some fibres ranked higher for butyrate (galacto-oligosaccharides) or propionate (rhamnose), choosing a substrate to enhance the total amount of a particular SCFA was difficult.
Different fibres and their breakdown products are fermented by individual species or by consortia of bacteria. Primary degraders initially depolymerise polysaccharides forming smaller units that can either be utilised by themselves or processed by secondary degraders(Reference Tomlin, Read and Edwards3). The metabolic products of this process can go through various stages of transformation. Additionally, some bacteria can metabolise products such as acetate and lactate from primary degraders and convert them into other molecules such as butyrate(Reference Morrison, Mackay and Edwards65). Intestinal absorption of SCFA promotes water absorption(Reference Ruppin, Bar-Meir and Soergel66), therefore, if SCFA production is spread throughout the colon this may aid in stool softness(Reference Edwards and Eastwood37). The process of fermentation also alters the physical structure of fibres and can reduce the water-holding capacity of readily fermented fibres such as pectin and guar. As a result, they have very little effect on stool output and are mostly fermented in the proximal colon. The production of SCFA can impact bacterial composition; beneficial bacteria such as lactobacilli and bifidobacteria prefer a more acidic pH whereas less desirable bacteria tend to prefer a slightly neutral or alkali pH.
There is growing interest in the health effects of individual SCFA through their potential roles in modulating metabolic health, gut barrier function, glucose homoeostasis, immune function, obesity and appetite regulation(Reference Chambers, Preston and Frost67). Butyrate is the main fuel for colonocytes and has potential anti-inflammatory and anti-carcinogenic roles(Reference Cao, Zhang and Han68). Acetate and propionate may influence satiety through the stimulation of G-protein coupled receptors in the colon (FFAR2) promoting the release of the hormones PYY and GLP-1(Reference Bolognini, Dedeo and Milligan34). Acetate can also increase hepatic lipogenesis whereas propionate may inhibit cholesterol and lipid synthesis, increase gut cell proliferation and increase insulin sensitivity(Reference Morrison and Preston35).
The polyphenols often associated with fibre are metabolised by the bacteria to a range of phenolic acids such as 3,4-dihydroxyphenylacetic acid from quercetin, which may influence key functions in the body such as inhibition of platelet aggregation(Reference Rechner and Kroner57), suppression of pro-inflammatory cytokines(Reference Monagas, Khan and Andrés-Lacueva58) and inhibition of protein glycation(Reference Vlassopoulos, Lean and Combet55,Reference Pashikanti, de Alba and Boissonneault59) . Many other bacterial products (e.g. phenols and cresols and hydrogen sulphide) which may be both positive or harmful in the body may be affected by fibre fermentation(Reference Verbeke, Boobis and Chiodini69) but their impact on health is not yet clear. Bacterial enzymes for some potential reactions may also need to be induced by exposure to particular substrates or bonds from recent dietary intake.
Conclusion
Variability in human guts and their microbiome may require a personalised approach
It is clear that dietary fibre and the gut microbiota are important moderators of gut health and the production of a range of molecules that could influence the function of many body systems. However, it is difficult to establish which fibres should be eaten to gain the best overall impact on the microbiota and health. Inter- and intra-individual variability presents a significant challenge in nutritional science. Variability may be a considerable grievance to nutrition researchers trying to identify the effects of interventions, but it is increasingly evident that variation is an inevitable part of human studies. Individuals can have significantly different gut microbiota composition and background diets. These differences may be related to age and sex(Reference Alkhaldy, Edwards and Combet70,Reference Resch, Parikh and Austria71) . The response to fibre and polyphenol intake also varies considerably between individuals(Reference Havlik, Marinello and Gardyne29). Individuals could also have inherent differences in gut physiology such as gut muscle tone, receptor profiles and pain perception leading to differences in tolerance of fibre doses and gas production, and/or digestive symptoms. Bacterial metabolite profiles differ among individuals fed the same diets and subsequent physiological responses to those metabolites may also vary(Reference Havlik, Marinello and Gardyne29,Reference Rinninella, Raoul and Cintoni72) . The factors which determine this individual variability need further research. However, this may indicate the need for a more personalised approach in identifying the best dietary fibre and polyphenol sources and doses for promoting a healthy gut microbiota and those bioactive microbial products most likely to have a profound effect on human health throughout the human body and through the gut brain axis.
Financial Support
None.
Conflict of Interest
C. A. E. was chair of a working group on early colonisation of infant gut for ILSI Europe.
C. T. is a CTP PhD student supported/partially funded by Mondelez International (BBSRC grant award number: 2294514).
Authorship
The authors had sole responsibility for all aspects of preparation of this paper.