Book contents
- Frontmatter
- Contents
- Acknowledgments
- 1 Extreme environments: What, where, how
- 2 Properties of dense and classical plasma
- 3 Laser energy absorption in matter
- 4 Hydrodynamic motion
- 5 Shocks
- 6 Equation of state
- 7 Ionization
- 8 Thermal energy transport
- 9 Radiation energy transport
- 10 Magnetohydrodynamics
- 11 Considerations for constructing radiation-hydrodynamics computer codes
- 12 Numerical simulations
- Appendix I Units and constants, glossary of symbols
- Appendix II The elements
- Appendix III Physical properties of select materials
- References
- Further reading
- Index
- References
Further reading
Published online by Cambridge University Press: 05 November 2013
- Frontmatter
- Contents
- Acknowledgments
- 1 Extreme environments: What, where, how
- 2 Properties of dense and classical plasma
- 3 Laser energy absorption in matter
- 4 Hydrodynamic motion
- 5 Shocks
- 6 Equation of state
- 7 Ionization
- 8 Thermal energy transport
- 9 Radiation energy transport
- 10 Magnetohydrodynamics
- 11 Considerations for constructing radiation-hydrodynamics computer codes
- 12 Numerical simulations
- Appendix I Units and constants, glossary of symbols
- Appendix II The elements
- Appendix III Physical properties of select materials
- References
- Further reading
- Index
- References
Summary
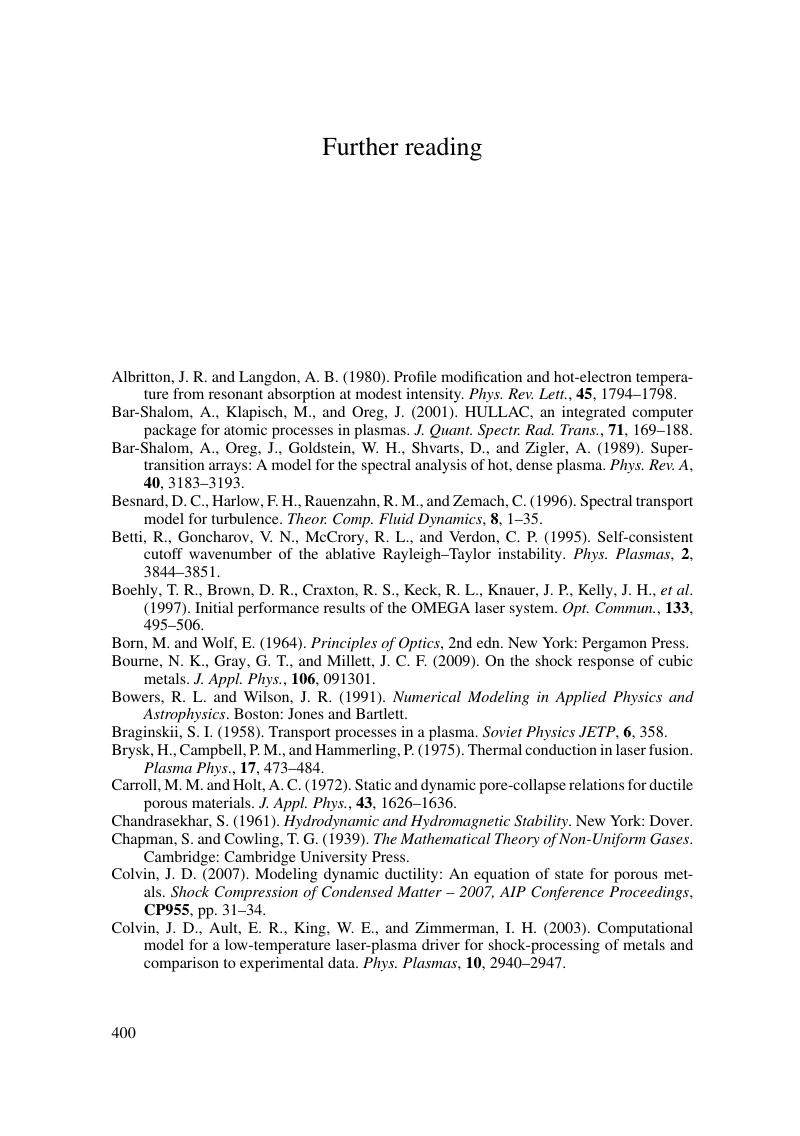
- Type
- Chapter
- Information
- Extreme PhysicsProperties and Behavior of Matter at Extreme Conditions, pp. 400 - 402Publisher: Cambridge University PressPrint publication year: 2013