6.1 Introduction
Palaeomagnetic dating methods are based on comparing magnetic information from materials or sequences, whose ages are at least partly unknown, with the geomagnetic chronology. The global Quaternary geomagnetic chronology is continuously updated, and currently includes 10 polarity reversals and at least 18 validated geomagnetic excursions that serve as chronological markers for sedimentary and volcanic sequences. In addition, short-term secular variations of the past several millennia can be used for the Holocene. Here, we review the principles underlying palaeomagnetic chronology with emphasis on Quaternary rocks, sediments, and archeo-logical substances. We summarize a number of successful applications of palaeomagnetic dating in the Levant, and provide insight into future possibilities for Quaternary palaeomagnetism.
6.1.1 General Palaeomagnetism
The Earth's magnetic field is constantly changing on time scales of decades to millions of years. The effort to reconstruct past geomagnetic variations has been motivated mainly by a geophysical interest in understanding the behaviour of the Earth's magnetic field, its origin, and its impact on the Earth's atmosphere and biosphere. The outcome of this research is a fairly robust geomagnetic record that can be used as an independent chronological reference framework.
To apply palaeomagnetism for dating, a robust geomagnetic chronology needs to be established first, by compiling the global palaeomagnetic database into three independent variation records: (1) reversals, (2) excursions, and (3) short-term (secular) changes in direction and intensity. Then, on the basis of comparison with the appropriate reference chronology, the magnetic polarity, direction, and intensity of materials or sequences are used to obtain age estimates.
Palaeomagnetism is a powerful chronological tool that has been used for decades (Sternberg Reference Sternberg, Taylor and Aitken1997; Singer Reference Singer2014). Yet it is important to emphasize that it involves a series of experimental procedures that require several stages of interpretation. Also, it is based on the reference geomagnetic chronology to date. Hence, when adopting palaeomagnetism, an understanding of how it works is required. Here we review the principles underlying palaeomagnetic methods, so that readers may adopt a realistic and critical view, and can adequately design palaeomagnetic dating applications in their research. In Section 6.2 we briefly explain how different materials record magnetic information. In Section 6.3 we outline the basic palaeomagnetic laboratory procedures. In Section 6.4 we review the up to date geomagnetic chronologies. Finally, in Section 6.5 we review the application of palaeomagnetic dating in selected geological and archeological studies of the Levant.
6.2 Principles of Natural Magnetic Recording
Ferromagnetic minerals (for simplicity, we group different types of magnetic minerals – with ferro-, ferri-, anti-ferro, canted-, and defect-magnetism – under the one term ferromagnetic) are the fundamental building blocks of magnetic recording. Ferromagnetic minerals have an intrinsic spontaneous magnetization, which makes them act as small-scale ‘compass needles’. The ferromagnetic minerals that are relevant to palaeomagnetism are those whose magnetization is locked in space at room temperature. The most abundant groups of ferromagnetic minerals are iron oxides: magnetite (Fe3O4), hematite (Fe2O3), and maghemite (γ-Fe2O3); iron sulfides: greigite (Fe3S4), pyrrhotite (Fe1-xSx), and iron oxyhydroxides (e.g. goethite, α-FeOOH). These groups can appear in their pure form or with substitutes. They are so abundant that they can be found virtually everywhere: in rocks, sediments, soils, dust, and various archeological materials.
The mechanisms by which ferromagnetic minerals record and retain magnetic information (called hereafter remanent magnetization) can be classified into: thermomagnetic (TRM – magnetization acquired by cooling), depositional (DRM – physical settling of magnetic particles in sedimentary environments), and chemical (CRM – chemical growth of magnetic minerals). Figure 6.1 illustrates these mechanisms graphically. For further reading, see Dunlop and Özdemir (Reference Dunlop and Özdemir2001), and Tauxe (Reference Tauxe2010). TRM (Fig. 6.1a) is acquired in igneous rocks and fired archeological materials when ferromagnetic minerals are cooled through a critical temperature called the blocking temperature (TB). In TB, the spontaneous magnetization becomes locked in space, and a magnetization is gained in a direction parallel to the ambient field. DRM (Fig. 6.1b) can be acquired in marine, lake, and aeolian environments. DRM occurs when ferromagnetic particles align in the direction of the ambient field before settling. CRM (Fig. 6.1c) is acquired when new ferromagnetic grains grow and possess magnetization. In addition to the mechanisms shown in Fig. 6.1, other processes contribute to the net magnetization of a material. The stability (or the ‘decay’ time) of the magnetization, which depends on the age, mineralogy, and size of the particles, may lead to an acquisition of a ‘viscous’ magnet-ization (VRM). Various physical and chemical alterations may also distort, erase, and even re-magnetize an existing magnetic signal through time.

Figure 6.1 Schematic illustration of the three main mechanisms of magnetic remanence acquisition.
To exemplify the concept and complexity of palaeomagnetic recording, we consider as case studies three lithologies relevant to Quaternary sequences: a lava flow, a lake varve, and a buried soil. Ideally, the lava flow would have a TRM recorded on the day the basalt cooled, the lake varve would have a pure DRM that became locked in over a season, and the buried soil would have a CRM acquired over a specific polarity chron (see below for definitions of chrons). In these examples, the original primary magnetization is tied directly to the age of the material, and is referred to hereafter as the characteristic remanent magnetization (ChRM). In practice, the ChRM is often overprinted by many magnetic signals postdating the age of the ChRM. For example, the basalt can acquire ‘viscous’ magnetization (VRM), can be demagnetized by chemical and physical weathering (CRM), and might even be struck by lightning (totally erasing the original TRM); the lake varve can lose its DRM by bioturbation, oxidation, dissolution, and alteration (Fig. 6.1b), and can be masked by new ferromagnetic minerals growing in the sedimentary column (i.e. CRM); the buried soil can have a ‘soft’ and unstable magnetization that decays with time or becomes masked by new CRM that continues to form as the soil ages.
6.3 Basic Palaeomagnetic Procedures
Given the complexity of the natural magnetic acquisition (Section 6.2), there are some questions that should be properly addressed before one uses palaeomagnetism: (1) ‘Which minerals carry the magnetic signal?’, (2) ‘What are the processes by which the magnetic signal was acquired?’, and (3) ‘When was the magnetic signal acquired?’ Also, obviously, we need a methodology for measuring the ChRM and isolating it from all possible overprints. Assessing these questions is the art of palaeomagnetic lab work.
There are different parameters and levels of palaeomagnetic information that can be retrieved by palaeomagnetic analyses. In some cases, the desired information might simply be the polarity of the ancient field (‘Normal’ or ‘Reverse’; Section 6.4). In other cases, the aim might be to recover the precise direction and/or intensity of the ancient field.
Almost all types of palaeomagnetic investigations require isolation of the ChRM. This is done by progressively demagnetizing the net magnetization (natural remanent magnetization, NRM) of the sample. Demagnetization can be done thermally, by heating the sample in zero-field conditions, or magnetically, by exposing the sample to a strong alternating magnetic field (AF). After each demagnetization step, a portion of the NRM vector is lost, and the x, y, z components of the remaining vector are measured with a high-sensitivity magnetometer. Consecutive measurements of the remaining NRMs are plotted on an orthogonal plot where the length of the magnetization vector is normalized to the length of the initial NRM. An orthogonal plot that appears as a straight line converging toward the origin (Fig. 6.2) means that the direction of the vector remained the same after all the demagnetization steps. In this case the ChRM is calculated through principal component analysis (Kirschvink Reference Kirschvink1980). A more complicated orthogonal plot should be interpreted with care. A robust demagnetization procedure would include both AF and thermal techniques. An example is shown in Fig. 6.2.
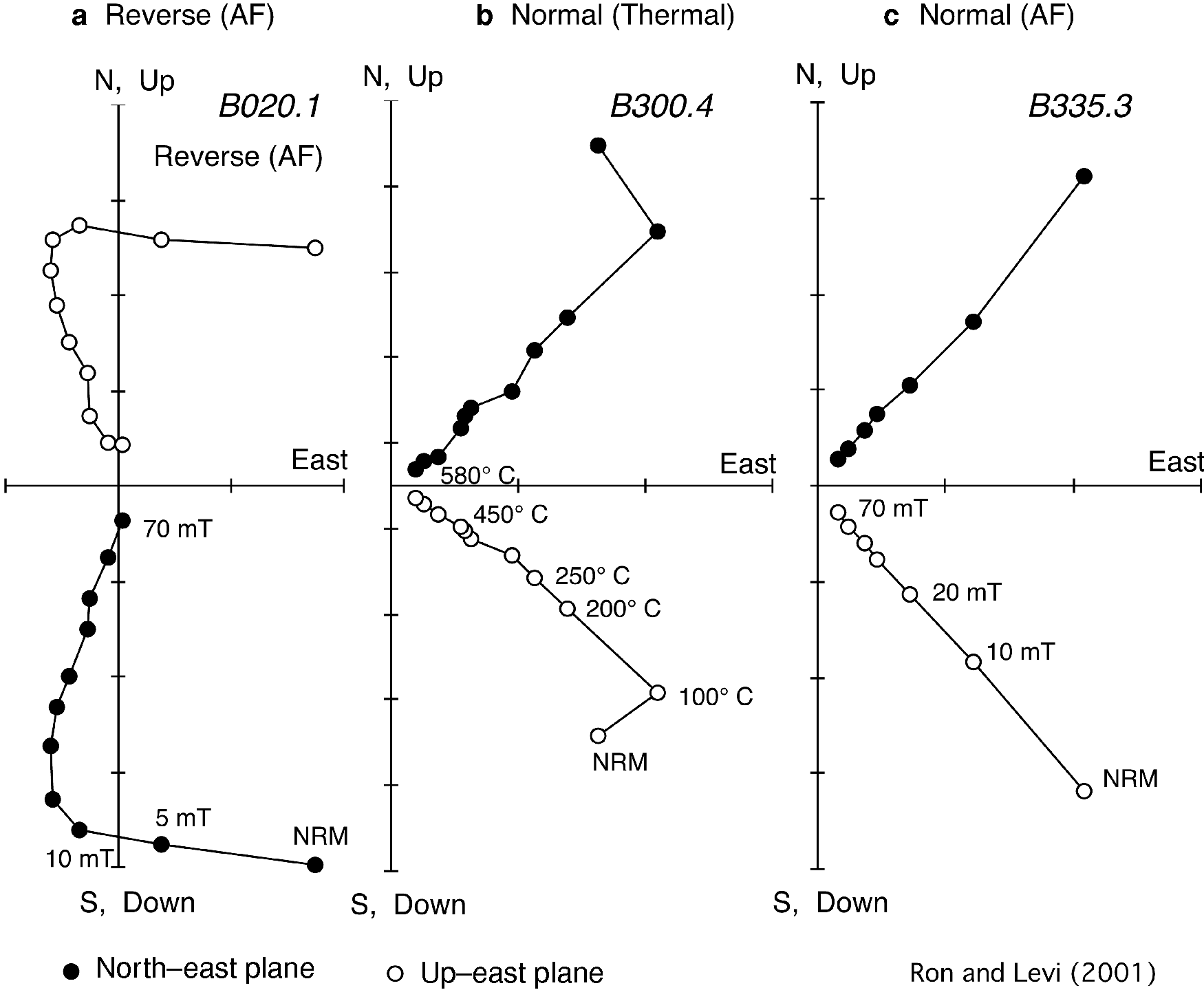
Figure 6.2 Examples of demagnetization experiments displayed on orthogonal plots, modified from a study of Erq el Ahmar Formation by Ron and Levi (Reference Ron and Gvirtzman2001). a: AF demagnetization revealing stable Reverse polarity (up and south; see Fig. 6.3); b and c: thermal and AF experiments on sister specimens showing similar Normal polarity (down and northeast).
The palaeomagnetic vector is described by the declination (deviation from the geographic north), and inclination (the angle between the vector and the horizontal plane). The palaeomagnetic direction is usually calculated as a mean of several samples collected from the same ‘site’, where a ‘site’ is a unit representing the same point in time, e.g. a lava flow or a particular horizon in a sedi-mentary sequence. The site palaeomagnetic mean is calculated via Fisher statistics (Fisher Reference Fisher1953). Similarly, one can calculate the palaeomagnetic mean of a locality by a Fisher mean of several sites.
Estimating the intensity of the ancient field (palaeointensity) is by far more complicated than estimating the palaeomagnetic direction. The experimental principles of palaeointensity are beyond the scope of this chapter (see Valet 2003; Tauxe & Yamazaki Reference Tauxe, Yamazaki and Kono2007; Shaar et al. Reference Shaar, Ron and Tauxe2010; Shaar & Tauxe Reference Shaar and Tauxe2013), but it is the basis of a relatively new archeomagnetic chronological tool for the Holocene (Section 6.4.2).
As mentioned above, a robust palaeomagnetic analysis would not be complete without assessment of the ‘which’, ‘what’, and ‘when’ questions on the ancient magnetization. Yet this is far from being a trivial task. Above all, the fundamental data to consider are the geological setting, the detailed lithology, and the history of the system (formation and post-depositional). Then, there is a wide range of magnetic experimental procedures and microscopic observations that can assist in characterizing the magnetic mineralogy, grain size distributions, magnetization stability, and the origin of the magnet-ization (Dunlop & Özdemir Reference Dunlop and Özdemir2001; Tauxe Reference Tauxe2010; Liu et al. Reference Liu, Roberts and Larrasoana2012). There is no simple recipe for this so-called ‘rock-magnetic analysis’, and its scope depends on the specific requirements of the study. Also, interpreting rock-magnetic data is not always simple. It is likely that some uncertainties about the rock-magnetic properties of the material will still remain open after rigorous analysis. However, we stress that palaeomagnetic interpretations based on inadequate assessment of the source, the age, and the stability of the magnetization should always be regarded with extreme caution.
6.4 Geomagnetic Chronology
6.4.1 Geomagnetic Polarity/Instability Time Scales
Geomagnetic reversals are one of the most prominent chronological markers in the Quaternary. During a reversal, the polarity of the field rapidly switches between N/R states, where N (Normal) stands for northerly oriented field and R (Reverse) stands for southerly oriented field. The duration of a reversal is less than 104 years, perhaps even less than 103 years (Valet & Fournier Reference Valet and Fournier2016). The reversal chronology, known as the ‘Geomagnetic Polarity Time Scale’ (GPTS), started to be developed in the early 1960s (e.g. Cox et al. Reference Cox, Doell and Dalrymple1964), when Quaternary GPTS included only two polarity ‘chrons’ and one short-term event. The chrons were initially named after pioneers in geomagnetism (Brunhes, Matuyama, and Gauss; Cox et al. Reference Cox, Doell and Dalrymple1964; Fig. 6.3). With time, more reversals were discovered, and the nomenclature of GPTS became too complicated to maintain. The currently accepted terminology divides the Quaternary into chrons and subchrons (Cande & Kent Reference Cande and Kent1995; Gee & Kent Reference Gee, Kent and Kono2007). Some of the subchrons are still known by their initial names (Jaramillo, Cobb Mountain, Olduvai, Reunion/Feni), and this nomenclature remains in widespread use. We note that GPTS terminology in the published literature, especially for short-term events in the Matuyama, such as Cobb Mountain, Gilsa, and Reunion, is not always consistent (for further reading see Gee & Kent Reference Gee, Kent and Kono2007; Tauxe Reference Tauxe2010; Singer Reference Singer2014). As the age accuracy of the GPTS is of great importance in Quaternary research, it is continuously updated using new high-quality data from sedimentary, oceanic, and volcanic sources (Gee & Kent Reference Gee, Kent and Kono2007; Gradstein et al. Reference Gradstein, Ogg, Schmitz and Ogg2012; Singer Reference Singer2014). Recently published Quaternary GPTSs differ slightly from each other and display some minor discrepancies, reflecting that the precise chronology is a work in progress.

Figure 6.3 The Quaternary Geomagnetic Polarity Time Scale. The chrons and subchrons shown follow Singer (Reference Singer2014). Excursions validated by Laj and Channell (Reference Laj, Channell and Kono2007) and Roberts (Reference Roberts2008) are shown as arrows.
Geomagnetic excursions are additional important detectable chronological markers. Excursions are brief (<104 years) and substantial deviations of field direction from northerly/southerly oriented geomagnetic field (>45° declinational change; Laj & Channell Reference Laj, Channell and Kono2007), accompanied by a significantly low field intensity (Roberts Reference Roberts2008). As excursions are short events, they are hard to detect, and therefore there is controversy in the literature regarding some of the published Quaternary excursions. We show in Fig. 6.3 excursions validated by Laj and Channell (Reference Laj, Channell and Kono2007), and by Roberts (Reference Roberts2008).
6.4.2 Holocene Geomagnetic Secular Variations
A relatively new addition to the palaeomagnetic geochronology toolbox is regional curves of geomagnetic secular variations. Secular variations are short-term (<105 years) changes in the direction and intensity of the field. If a regional secular variation curve is established for a given time interval, then palaeomagnetic dating can be applied by comparing direction and/or intensity of material/sequences whose age is unknown with the reference secul-ar variation curve. While this concept is not new, its application requires robust and accurate reference curves for secular variations. We have compiled such a curve for the Levantine intensity using a range of well-dated archeological materials. This is a work in progress, and its initial version is illustrated in Fig. 6.4.

Figure 6.4 Archeomagnetic ages of young materials using palaeointensity. The Levantine Archaeomagnetic Compilation (LAC) is an in-progress effort to construct a new archeo- and geochronology for young fired archeological objects (R. Shaar & E. Ben-Yosef, unpublished). The grey stripe is palaeointensity results from a sample whose age is unknown. A comparison with LAC suggests an Iron-Age date. Similar declination/inclination curves for the Holocene may provide geochronology for young sediments.
6.5 Applications of Palaeomagnetic Geochronology in the Levant
Palaeomagnetic chronology has been applied in a number of Levantine sequences for which direct absolute age dating could not be accomplished, or as a complementary method. Here, we briefly review some successful applications of palaeomagnetic chronology in geological and archaeological contexts. Notably, the most densely sampled area is within Israel where the late Professor Hagai Ron established a laboratory in 1980 (Fig. 6.5).

Figure 6.5 Location map of the studies listed in Section 6.5.
6.5.1 Palaeomagnetic Dating of Geological Units
Volcanic Sections
In a pioneering piece of research, Freund et al. (Reference Freund, Oppenheim and Schulman1965) conducted one of the first worldwide palaeomagnetic chronostratigraphic applications, studying the Plio-Pleistocene volcanic field in Northern Israel. This volcanic field was later densely sampled for both palaeomagnetic and radiometric analyses (Ron et al. Reference Ron, Freund, Garfunkel and Nur1984, Reference Ron, Heimann and Garfunkel1992; Heimann & Ron Reference Heimann and Ron1993; Heimann et al. Reference Heimann, Steinitz, Mor and Shaliv1996; Hurwitz et al. Reference Hurwitz, Matmon, Ron and Heiman1999). Magnetostratigraphy was used by these authors to refine K–Ar ages of successive lava flows (e.g. Nahal Orvim, Nahal Ashaf), infer ages of fluvial units (e.g. Nahal Hazor), and correlate different sections in the Golan Heights and Korazim block (Heimann & Ron Reference Heimann and Ron1993). These two methods were combined by dating a few of the flows in each sequence, including the uppermost and the lowermost ones, and then using them as anchors for GPTS correlation of all flows. The volcanic field outside the Golan Heights was less studied. Vandonge et al. (Reference Vandonge, Vandervo and Raven1967) and Gregor et al. (Reference Gregor, Mertzman, Nairn and Negendan1974) investigated volcanic units in Syria and Lebanon. Abou-Deeb et al. (Reference Abou-Deeb, Otaki, Tarling and Abdeldayem1999) used palaeomagnetic directions and polarities to obtain a chronological differentiation between independent volcanic units in Syria.
Loess and Ancient Soils
Aeolian deposits and buried soils carry excellent magnetic signal. Yet their net magnetization is a complex combination of different DRM, post-depositional DRM (pDRM), and CRM mechanisms acting over a relatively long period (Evans & Heller Reference Evans and Heller2001; Liu et al. Reference Liu, Deng, Torrent and Zhu2007). Thus, magnetostratigraphy analysis of loess and buried soils should take into account possible palaeomagnetic delay (lock-in depth) of the order of 105 years. Despite this complexity, mag-netostratigraphy was successfully applied on different types of buried soils, loess, and Hamra soil in Israel: Evron Quarry (Ron et al. Reference Ron, Porat, Ronen, Tchernov and Horwitz2003), Revadim Quarry (Gvirtzman et al. Reference Gvirtzman, Wieder and Marder1999; Marder et al. Reference Marder, Gvirtzman and Ron1999), and the Ruhama badlands (Laukhin et al. Reference Laukhin, Ronen and Pospelova2001; Ron & Gvirtzman Reference Ron and Gvirtzman2001).
Calcretes
Mashiah et al. (Reference Mashiah, Greenbaum, Zilberman, Ron and Ronen2009) recognized that calcretes (consolidated carbonate soil horizons) carry stable CRM. They used this phenomenon to date successive sections of calcretes in the base of Mt Carmel via magnetostratigraphy as a means to put temporal constraints on the development of the Western Carmel escarpment. Zilberman et al. (Reference Zilberman, Ron and Shaar2011) and Zilberman (Reference Zilberman2013) used a similar approach to investigate calcretes overlying faults in Western Galilee and Modiin to constrain termination of the tectonic activity. Greenbaum and Zilberman (Chapter 47 of this volume), using similar approaches, were able for the first time to decipher a Plio-Pleistocene chronology of alluvial and coastal units in the northwestern Negev. Matmon et al. (Reference Matmon, Katz and Shaar2010) applied palaeomagnetic methods to investigate the temporal evolution of successive colluvial units in the Zurim escarpment, Bet-Kerem Valley.
Lacustrine Deposits
Palaeomagnetism of the thick lacustrine sequence of the Dead Sea Group has been extensively studied, including the Sedom, Erq el Ahmar, ‘Ubeidiya, Lisan, and Zeelim Formations (e.g. Matmon & Zilberman, Chapter 3; Bar-Yosef & Belmaker, Chapter 20; Stein et al., Chapter 8 of this volume). Key palaeomagnetic studies are reviewed here as examples.
Weinberger et al. (Reference Weinberger, Agnon and Ron1997) employed palaeomagetism for reconstructing the tectonic evolution of the Sedom diapir. In their analysis, they isolated a secondary normal-polarity magnetization associated with Brunhes polarity. This CRM postdates the main tilt and constrains the termination of diapir emplacement.
The magnetostratigraphy of the Erq el Ahmar (EEA) Formation was investigated in three different works (Braun et al. Reference Braun, Ron and Marco1991; Ron & Levi Reference Ron and Gvirtzman2001; Davis et al. Reference Davis, Matmon, Fink, Ron and Niederniann2011). There are only minor differences between the palaeomagnetic profiles, and they all agree well with the initial profile of Braun et al. (Reference Braun, Ron and Marco1991). Yet the palaeomagnetic age interpretations given in the above publications are different. This exemplifies the dependency of magnetostratigraphy on absolute dates at tie points. Using an estimated biostratigraphic age of 1.5–2 Ma, Braun et al. (Reference Braun, Ron and Marco1991) correlated the EEA Normal polarity to either Olduvai or Reunion subchrons. Later, Ron and Levi (Reference Ron and Gvirtzman2001) obtained a more detailed inclination profile, concluding that the subchron is more likely Olduvai. Recently, Davis et al. (Reference Davis, Matmon, Fink, Ron and Niederniann2011) obtained 11 cosmogenic burial ages of 3.5–5.3 Ma, pushing the mag-netostratigraphy sequence to the Gauss or Gilbert chrons. Mag-netostratigraphy analysis of the younger ‘Ubeidiya Formation, located a few kilometres north of Erq el Ahmar, yielded four reversal events. Given faunal constraints of about 1.4 Ma, Sagi (Reference Sagi2005) provided several possible age interpretations, all within the Matuyama chron.
Sections of the late Pleistocene Lisan Formation near Nahal Prazim, Masada, and southern Sea of Galilee (Lake Kinneret) (Ohalo; Nadel, Chapter 33 of this volume) were studied for palaeomagnetism for recovering short-term secular variations of the geomagnetic field (Marco et al. Reference Marco, Ron, McWilliams and Stein1998, Reference Marco, Ron, McWilliams, Stein, Tarling and Turner1999; Marco Reference Marco2002). Using AF and thermal demagnetization procedures, they isolated the palaeomagnetic direction, and obtained detailed secular variation profiles revealing two excursions in the Peratzim section (Marco et al. Reference Marco, Ron, McWilliams and Stein1998) and one excursion in Ohalo (Marco Reference Marco2002). Similar investigations targeted the Holocene in Lake Kinneret (Thompson et al. Reference Thompson, Turner, Stiller and Kaufman1985) and in the Dead Sea exposure and core data (Segal Reference Segal2003; Frank et al. Reference Frank, Nowaczyk and Negendank2007a, Reference Frank, Nowaczyk and Negendank2007b).
Despite the success of the studies above, questions regarding the stability and origin of the magnetization in the Dead Sea sediments were not raised until recently (Ron et al. Reference Ron, Nowaczyk, Frank, Marco and Mcwilliams2006, Reference Ron, Nowaczyk and Frank2007; Frank et al. Reference Frank, Nowaczyk and Negendank2007a, Reference Frank, Nowaczyk and Negendank2007b; Nowaczyk Reference Nowaczyk2011). Using thorough rock-magnetic and microscopic investigation of the Lisan and Zeelim Formations, they deciphered complex histories of magnetic acquisition. Ron et al. (Reference Ron, Nowaczyk, Frank, Marco and Mcwilliams2006) suggested a three-stage model for the complicated magnetization: (1) DRM acquisition of fine magnetite particles, (2) CRM acquisition of greigite accompanying the dissol-ution of the primary magnetite, and (3) oxidation of the greigite following outcrop exposure. This model suggests that palaeomagnetic data from Dead Sea rift lacustrine deposits should be cautiously examined.
Only few sedimentary Quaternary sequences outside the Dead Sea basin were studied using palaeomagnetic methods. Frank et al. (Reference Frank, Schwab and Negendank2002) investigated Holocene palaeomagnetic secular variations in Birkat Ram, the Golan Heights. Develle et al. (Reference Develle, Gasse and Vidal2011) used inclination anomalies derived from the Yammouneh basin core, Lebanon, to establish a palaeomagnetic chronology based on excursion markers.
6.5.2 Archaeomagnetism
Over the past several decades, Levantine archeological research has involved palaeomagnetic research, predominantly for constraining ages of archeological finds. The GPTS records obtained from sedimentary and volcanic sequences associated with archeological material have been used to estimate ages of several important prehistoric sites, some of which are key for understanding early human evolution and migration routes. The age of ‘Ubeidiya (Bar-Yosef & Belmaker, Chapter 20 of this volume), the earliest site in the Levant and one of the earliest outside Africa, was further constrained by palaeomagnetic study of the ‘Ubeidiya sequence to specific time intervals (1.55–1.2 Ma or 1.2–1 Ma; Sagi Reference Sagi2005). It has been suggested that a nearby site, Erq el Ahmar, is even earlier (>2 Ma; see above and Davis et al. Reference Davis, Matmon, Fink, Ron and Niederniann2011; Matmon & Zilberman, this volume). However, the magnetostratigraphy has no palaeoanthropological material and the association of the Erq el Ahmar Formation with such material is debated. Another key prehistoric site is Gesher Benot Ya'aqov (GBY) (Goren-Inbar, Chapter 21 of this volume). The site, an accumulation of artefacts and ecofacts along a thick sedimentary sequence, was deposited through the Brunhes/Matuyama transition (∼780 ka; Goren-Inbar et al. Reference Goren-Inbar, Belitzky and Verosub1992, Reference Goren-Inbar, Feibel and Verosub2000). Similar to ‘Ubeidiya, GBY is also in the Rift Valley of northern Israel, along which hominin expansion northward probably took place. The palaeomagnetic stratigraphy contributes to our understanding of this process, and possibly also to the question of the timing of the first deliberate use of fire (see Goren-Inbar et al. Reference Goren-Inbar, Alperson and Kislev2004). Other Lower Palaeolithic excavations at which magnetostratigraphy has successfully been applied are Evron Quarry (Middle Acheulian, Ron et al. Reference Ron, Porat, Ronen, Tchernov and Horwitz2003), Bizat Ruhama (Laukhin et al. Reference Laukhin, Ronen and Pospelova2001; Ron & Gvirtzman Reference Ron and Gvirtzman2001), Late Acheulian Revadim Quarry (Gvirtzman et al. Reference Gvirtzman, Wieder and Marder1999; Marder et al. Reference Marder, Gvirtzman and Ron1999) and Kefar Menachem (lower Palae-olithic, Malinsky-Buller et al. Reference Malinsky-Buller, Barzilai and Ayalon2016). However, considering the importance of the Levant in early prehistory and the substantial number of key sites subjected to systematic excavations, the number of reported sites applying palaeomagnetism is surprisingly low.
While the GPTS is the basis for constraining the age of prehistoric sites, secular variations records are used for dating younger sites (usually not earlier than the Pottery Neolithic, the time when ceramic technology was introduced). However, the application of the latter is still limited as the resolution of the published records is currently low (Fig. 6.4). Improvement of resolution and accur-acy of the reference curves for the Holocene is an ongoing effort (Figs. 6.4, 6.5). Such efforts are taking advantage of the rich archeological landscape of the Levant, based on well-dated heat-impacted archeological materials, e.g. Ben-Yosef et al. (Reference Ben-Yosef, Tauxe and Ron2008b), Gallet et al. (Reference Gallet, Genevey, Le Goff, Fluteau and Ali Eshraghi2006), Shaar et al. (Reference Shaar, Ben-Yosef and Ron2011). Recently, the availability of the Levantine Archaeomagnetic Compilation (LAC) data consisting primarily of intensity values was instrumental in constraining the age of important archeometallurgical sites, some of which are considered the earliest in the Levant if not anywhere. While some of these results corroborate the early age of smelting in the southern Levant (Ben-Yosef et al. Reference Ben-Yosef, Ron and Tauxe2008a), others (Ben-Yosef et al. Reference Ben-Yosef, Tauxe and Levy2010) contradict proposals of Neolithic copper smelting in the region. As the resolution of the LAC improves, its application in young archaeological contexts will become more effective, with potential to become a potent dating tool in the research of this archaeologic-ally rich region.
Acknowledgements
Working on this review exposed us to a substantial amount of published and unpublished data produced by the meticulous research of our teacher and mentor, the late Professor Hagai Ron, who passed away in 2012 after a short battle with a vicious disease. Hagai was one of the worldwide pioneers in applying palaeomagnetism. His profound and longstanding impact on the palaeomagnetic research of the Levant goes far beyond this review chapter.
We would like to thank Amotz Agnon, Shmulik Marco, Ofer Marder, and Yehouda Enzel for helpful comments and reviews that substantially improved this chapter.