Introduction
The impact of Late-glacial and Holocene climate change on the hydrology and vegetation of the central Andes has been researched for decades but continues to produce a significant amount of debate (e.g. Reference BakerBaker and others, 2001; Reference GrosjeanGrosjean, 2001; Reference Quade, Rech, Betancourt and LatorreQuade and others, 2001; Reference Mourguiart and LedruMourguiart and Ledru, 2003). Because of its complex topography, diverse ecosystems and sensitive circulation regime (Reference Zhou and LauZhou and Lau, 1998; Reference GarreaudGarreaud, 1999, Reference Garreaud2000; Reference VuilleVuille, 1999; Reference Garreaud, Vuille and ClementGarreaud and others, 2003), the region does not respond uniformly to climate change, and thus many paleoenvironmental records are contradictory or asynchronous (e.g. Reference AbbottAbbott and others, 2003). In addition, questions remain as to the centennial- to millennial-scale forcing mechanisms that are responsible for these changes (Reference Hillyer, Valencia, Bush, Silman and Steinitz-KannanHillyer and others, 2009). More temporally long and high-resolution records are needed from the region to reconstruct an accurate paleovegetational history. This is especially true for records that contain biological proxies (pollen, phytoliths, macrofossils, etc.) because vegetation change is best interpreted through direct evidence. However, most pollen, phytolith and macrofossil studies conducted in the central Andes have been based on lake/bog sediments (Reference Hansen, Wright and BradburyHansen and others, 1984, Reference Hansen, Seltzer and Wright1994; Reference Hansen and RodbellHansen and Rodbell, 1995; Reference Chepstow-Lusty, Frogley, Bauer, Bush and HerreraChepstow-Lusty and others, 2003, Reference Chepstow-Lusty, Bush, Frogley, Baker, Fritz and Aronson2005; Reference Paduano, Bush, Baker, Fritz and SeltzerPaduano and others, 2003; Reference BushBush and others, 2005; Reference Hanselman, Gosling, Paduano and BushHanselman and others, 2005; Reference Weng, Bush, Curtis, Kolata, Dillehay and BinfordWeng and others, 2006; Reference Gosling, Bush, Hanselman and Chepstow-LustyGosling and others, 2008) or rodent middens (Reference HolmgrenHolmgren and others, 2001; Reference Latorre, Betancourt, Rylander and QuadeLatorre and others, 2002, Reference Latorre, Betancourt, Rylander and Quade2003) and many are limited by their temporal range, temporal resolution or both.
Tropical Andean ice cores contain long temporal records of past environmental conditions and can be analyzed at high resolution (Reference ThompsonThompson and others, 1995, Reference Thompson1998, Reference Thompson2006). The chemical and physical proxies commonly used in ice-core research reveal physical conditions of the past (e.g. paleotemperature, paleoprecipitation and paleocirculation), but do not provide direct evidence of vegetation change. Though fossil pollen grains are abundant and ubiquitous components of these ice cores (Reference Liu, Yao and ThompsonLiu and others, 1998, Reference Liu, Reese and Thompson2005, Reference Liu, Reese and Thompson2007), a systematic long-term pollen record has yet to be produced. Here we present a 25 ka ice-core pollen record from Nevado Sajama, western Bolivia. This research expands on previous pollen studies from the Sajama ice cap (Reference Liu, Reese and ThompsonLiu and others, 2005, Reference Liu, Reese and Thompson2007), which have shown pollen assemblages to be sensitive indicators of vegetation change at shorter (i.e. decadal to centennial) timescales. Our aim in this paper is to assess the sensitivity of this biological proxy in tropical ice cores over longer temporal ranges.
We begin this investigation with an overview of the study area and discuss the patterns of vegetation in the central Andes and the physical factors (e.g. precipitation, temperature, regional circulation regimes) that influence them. Next, in the methodology, we discuss the ice cores that were extracted from Sajama in 1997 and give a detailed description of our pollen-processing and -counting procedures. In the results and discussion, we present and discuss our findings from the pollen analysis on the Sajama ice core and show that pollen is a sensitive indicator of vegetation response at these longer temporal ranges. Finally, in the conclusion, we compare our findings at Sajama to the results of other regional paleoenvironmental records to highlight the patterns of climatic changes and vegetation response in the central Andes during the Late-glacial and Holocene periods. We also discuss the limitations of pollen as an ice-core proxy, as well as the unique contributions that pollen analysis adds to an ice-core paleoenvironmental record.
Study Area
Nevado Sajama (188060 S, 688530 W; Mt Sajama in Fig. 1) rises to 6542 m on the drier, western side of the Bolivian Altiplano. The vegetation of the Altiplano is generally classified as puna grassland (Reference TosiTosi, 1960). This vegetation type is not homogeneous throughout its range because it is affected by both latitudinal and elevational climatic gradients (Reference Reese and LiuReese and Liu, 2005a). The latitudinal gradient is largely a function of precipitation, with wetter conditions in the northeastern section of the Altiplano (precipitation >400mma–1) and drier conditions in the southwest (precipitation 100–400 mm a–1) (Reference Cabrera and TrollCabrera, 1968; Reference SeibertSeibert, 1998). Grasses (Poaceae) are more prevalent in the wetter sections of the puna along with a higher density of shrubs, while small shrubs (namely Asteraceae) dominate the drier portions (Reference SeibertSeibert, 1998; Reference Kuentz, Galan de Mera, Ledru and ThouretKuentz and others, 2007). Average annual precipitation at the base of Nevado Sajama is 316 mma–1 (Reference Hardy, Vuille, Braun and Keimig Fand BradleyHardy and others, 1998) and the vegetation composition near its base is typical of the dry puna ecosystem.
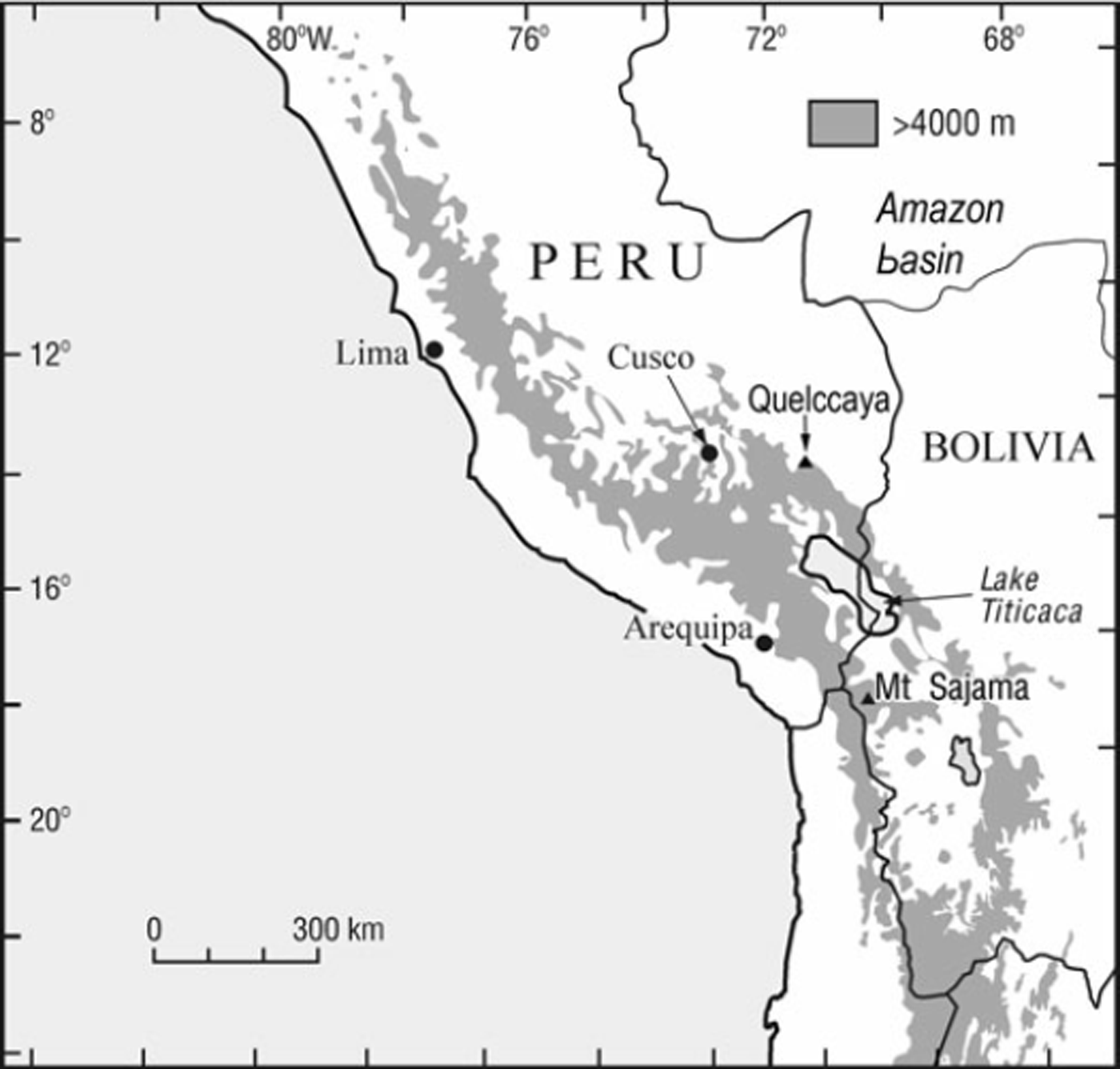
Fig. 1. Map of the central Andes showing the location of Nevado Sajama (adapted from Reference Reese, Liu and MountainReese and others, 2003).
The elevational facies of the puna have been defined in many ways (Reference TosiTosi, 1960; Reference Cabrera and TrollCabrera, 1968; Reference Cuatrecasas and TrollCuatrecasas, 1968; Reference Troll and TrollTroll, 1968; Reference GrafGraf, 1981; Reference Hansen, Wright and BradburyHansen and others, 1984; Reference Reese and LiuReese and Liu, 2005a). Reference Kuentz, Galan de Mera, Ledru and ThouretKuentz and others (2007) provide a useful summary of the previous definitions and propose their own detailed categorization. They subdivide the puna into three bioclimatic belts – the supratropical belt (3000–4000 m), the orotropical belt (4000–4800m) and the cryotropical belt (4800m to snowline) – all three of which can be found near or on the slopes of Nevado Sajama. The upper sections of the supratropical belt have high densities of shrubs, notably from the Asteraceae, Cactaceae, Fabaceae and Solanaceae familes. Small shrubs (especially Asteraceae) continue as a dominant plant in the orotropical belt, though during the wet season or in wetter years herbs (mainly Poaceae) may cover up to 75% of the soil. This orotropical belt also includes peat bog communities (or ‘bofedales’; Reference Baied and WheelerBaied and Wheeler, 1993) common to the Altiplano, and Polylepis woodlands. The peat bog communities grow in low-lying depressions or around sources of water. Here aquatics like Azolla are common, as well as Plantago, Distichia and members of the Cyperaceae and Apiaceae families (Reference Reese and LiuReese and Liu, 2005a; Reference Kuentz, Galan de Mera, Ledru and ThouretKuentz and others, 2007). At Sajama, thin stands of Polylepis tarapacana (Reference RadaRada and others, 2001) can be found on the slopes up to ∼5000m (Reference KesslerKessler, 1995). Only a small fraction of the original Andean Polylepis woodlands survive today (Reference Fjeldsa and KesslerFjeldsa and Kessler, 1996), though it is unclear whether climatic or anthropogenic factors through the Holocene contributed to their decline (Reference Hansen, Seltzer and WrightHansen and others, 1994). Finally, the cryotropical belt primarily consists of herbs that are highly adapted to intense physical weathering (Reference Kuentz, Galan de Mera, Ledru and ThouretKuentz and others, 2007). Here, Azorella (Apiaceae) is quite common, as well as members of the Caryophyllaceae, Malvaceae, Poaceae and Asteraceae families.
While the vegetation zones that surround the mountain are well understood, the source area for the pollen in the ice cap is less certain. Snow accumulation studies on Sajama show that only the summer wet-season precipitation/accumulation (December-March) is represented in the firn (Reference Hardy, Vuille and BradleyHardy and others, 2003). However, the loss throughout the year is primarily due to sublimation (Reference Hardy, Vuille and BradleyHardy and others, 2003), so dry-season pollen could potentially be present in the firn, as sublimation is thought to concentrate pollen in snow (Reference Liu, Reese and ThompsonLiu and others, 2007). Owing to the development of the South American summer monsoon (Reference Zhou and LauZhou and Lau, 1998), the prevailing winds during the accumulation months are decidedly out of the east and northeast (from the eastern Andean slopes and Amazonian lowlands) and therefore explain the high summer precipitation rates which represent over 80% of the annual total at Sajama (Reference Hardy, Vuille, Braun and Keimig Fand BradleyHardy and others, 1998; Reference Vuille, Hardy, Braun, Keimig and BradleyVuille and others, 1998). However, this circulation regime is regularly interrupted by El Nino Southern Oscillation (ENSO) events. During warm-phase (El Nino) conditions, decreased thunderstorm activity and the corresponding decrease in latent heating is thought to weaken the high-pressure cell over the Altiplano that produces this easterly flow (Reference SchwerdtfegerSchwerdtfeger, 1961; Reference Gutman and SchwerdtfegerGutman and Schwerdtfeger, 1965; Reference RamageRamage 1968; Reference DeanDean, 1971; Reference Kreuels, Fraedrich and RuprechtKreuls and others, 1975; Reference Rao and ErdoganRao and Erdogan, 1989; Reference Lenters and CookLenters and Cook, 1995). At Sajama, this change in circulation results in drier and windier conditions that are thought to increase sublimation, thus concentrating pollen (Reference Liu, Reese and ThompsonLiu and others, 2007). Modern pollen studies from Quelccaya ice cap, southern Peru (Fig. 1), corroborate these findings and show that the pollen assemblage within the ice is also affected by ENSO events (Reference Reese and LiuReese and Liu, 2005b). During El Nino, the pollen assemblage in the ice cap reflects the puna vegetation located on the Altiplano. However, during a normal (non-ENSO) year, the pollen assemblage reflects the dense Yungas (tropical forest) vegetation on the eastern Andean slopes and lowlands. This variability, coupled with the problems associated with the long-distance transport of pollen, makes the source region or regions of this pollen impossible to define. Thus, the pollen on Sajama provides a general, regional record of vegetation change for the central Andes. Nevertheless, the record is very useful as it comes from a unique archive, with a different depositional environment and pollen source region than previous Andean pollen records.
Methods
In 1997, two long cores were drilled to bedrock and extracted (Reference ThompsonThompson and others, 1998) from the summit of Nevado Sajama (Fig. 1). These cores (C-1 and C-2) were drilled 3 m apart and both were used for our palynological investigation. Although C-2 was used primarily for pollen sampling, samples were taken from C-1 in instances where sections of the primary core had already been heavily sampled. Dating control for the two long cores came from a combination of techniques including 3H (tritium) analysis, physical layer counts, calibrated 14C accelerator mass spectrometry (AMS) dates and stratigraphic d18O(atm) and d18O(water) correlations with the Greenland Ice Sheet Project 2 (GISP2) core from Greenland (Reference ThompsonThompson and others, 1998).
The pollen results for this record were obtained from 249 ice samples that cover the entire 132 m long Sajama ice core. Standard pollen-processing procedures for meltwater were used (Reference Liu, Yao and ThompsonLiu and others, 1998; Reference Reese and LiuReese and Liu, 2002). Each sample was first spiked with Lycopodium marker spores (Reference StockmarrStockmarr, 1971), then slowly evaporated down to 50 mL and transferred into centrifuge tubes. Next, each sample was treated with an acetolysis solution to remove cellulose, stained, and mounted on microscope slides for counting.
Samples range in resolution from 4 months to 550 years of accumulation. To standardize the record, the pollen data were grouped into ∼500 year time aggregates. Though it was impossible to create equal time aggregates throughout the core, all aggregates fall within ±50 years of the 500 year interval. This aggregation allows the entire record to be compared with minimal sampling bias.
A Nikon Optiphot microscope (at 400x magnification) was used for pollen and spore identification. All pollen and spores were counted until a total of 300 grains was obtained. The identifications were verified using pollen keys (Reference Van der Hammen and GonzalezVan der Hammen and Gonzalez, 1960; Reference HeusserHeusser, 1971; Reference Markgraf and D’AntoniMarkgraf and D’Antoni, 1978; Reference Roubik and MorenoRoubik and Moreno, 1991; Reference Colinvaux, De Oliveira and Moreno PatinoColinvaux and others, 1999) and the pollen reference slide library at Louisiana State University. Charcoal particles were counted and are reported, regardless of size, but the majority had maximum diameters of <50mm. Concentrations and percentages were calculated with TILIA software and TGView 2.0 was used to generate the pollen diagram (Fig. 2). The zones on Figure 2 are based on our interpretation of the pollen concentration and percentage data, but the titles for each zone follow the nomenclature of previous published studies on the Sajama ice core (Reference ThompsonThompson and others, 1998).

Fig. 2. Pollen diagram from Nevado Sajama.
Results and Discussion
Late-glacial stage
From 25 to 15 kaBP, pollen was absent from the record, or present in only small amounts (<8 grains per sample; Fig. 2). This lack of pollen probably reflects very sparse vegetation due to the extremely cold and wet conditions (Reference Gosling, Bush, Hanselman and Chepstow-LustyGosling and others, 2008), as well as reduced atmospheric partial CO2 pressure (Reference Boom, Mora, Cleef and HooghiemstraBoom and others, 2001) on the Altiplano during the Late-glacial stage (LGS). Low CO2 pressure has been shown to reduce/slow plant growth, thus potentially reducing the amount of pollen dispersed from these plants. The cordillera bordering the Altiplano was heavily glaciated during this period, which greatly reduced the local plant communities. High accumulation rates during this period would also work to dilute pollen concentrations in the ice core. Regardless, the near absence of pollen in this section makes any reliable interpretation of the paleovegetation very difficult.
Interstadial
At 15.5 ka BP, the oxygen-isotope data from Sajama indicate the beginning of a short period of warmer and possibly drier conditions that lasted until ∼14kaBP (Reference ThompsonThompson and others, 1998). Reference VuilleVuille and others (2003) have shown that enriched O1 8 values at Sajama are negatively correlated with precipitation (r=-0.66) and positively correlated with temperature (r=0.88). At ∼15kaBP, more pollen is found in the Sajama record and reflects the transition out of LGS conditions. Pollen concentrations range from 279 to 944 grains L–1 and the assemblage consists primarily of Poaceae (grass) and Asteraceae. Though the increase in pollen from these families indicates the postglacial expansion of the puna vegetation community, the distribution was likely still constricted and the cover was probably very sparse. Polylepis pollen also emerges in small quantities and remains a regular taxon throughout the rest of the record. The sporadic presence of Podocarpus and Myrica is likely due to long-distance transport.
This interstadial period closely matches the Bølling– Allerød/Guantiva interstadial event that has been recorded in several South American lake sediment records using a variety of chemical and biological proxies (Reference Van Geel and Van der HammenVan Geel and Van der Hammen, 1973; Reference Van der HammenVan der Hammen, 1974; Reference Helmens and KuhryHelmens and Kuhry, 1986; Reference Van der Hammen and HooghiemstraVan der Hammen and Hooghiemstra, 1995; Reference BakerBaker and others, 2001). However, there is some disagreement among the records as to the moisture characteristics of this event. Records from northern South America show this to be a warm and wet event (Reference Van der HammenVan der Hammen, 1974; Reference Helmens and KuhryHelmens and Kuhry, 1986), while records from the Altiplano show this to be a time of decreased precipitation (Reference ThompsonThompson and others, 1998; Reference BakerBaker and others, 2001; Reference Paduano, Bush, Baker, Fritz and SeltzerPaduano and others, 2003). The pollen evidence in this section of the ice core needs to be interpreted with caution due to low concentration numbers, but may shed additional light on the question of moisture availability during this period.
Several paleoecological studies from the Altiplano have suggested that increases in Poaceae and decreases in Asteraceae indicate wetter conditions (and vice versa) (Reference Betancourt, Latorre, Rech, Quade and RylanderBetancourt and others, 2000; Reference Latorre, Betancourt, Rylander and QuadeLatorre and others, 2002, Reference Latorre, Betancourt, Rylander and Quade2003; Reference Reese, Liu and MountainReese and others, 2003; Reference Liu, Reese and ThompsonLiu and others, 2005; Reference Reese and LiuReese and Liu, 2005a). In rodent middens and surface soils, the use of the Poaceae/Asteraceae ratio as a proxy for moisture conditions may be complicated by differential pollen preservation, but this limitation does not apply to ice-core samples. The Poaceae/Asteraceae proxy follows a real precipitation-driven vegetation gradient on the Altiplano, in which grasses dominate in the northern (wetter) sections of the region while Asteraceae shrubs dominate in the drier southern sections (Reference SeibertSeibert, 1998; Reference Kuentz, Galan de Mera, Ledru and ThouretKuentz and others, 2007). This ecological relationship forms the basis for our interpretation of the pollen record.
As temperatures warmed at the start of this short interstadial and pollen concentrations begin to rise, grasses dominate the record. We interpret this as a transitional time when the wet conditions during the glacial stage persisted for a few hundred years while temperatures ameliorated. However, toward the end of this period, Asteraceae began to dominate and was coupled with dryness indicators such as increases in Amaranthacae and Ephedra. During this period there is also a decrease in Polylepis, which Reference GrafGraf (1981) linked to drier conditions. Therefore, based on the pollen evidence, we interpret this period to have been both warmer and drier.
Deglacial climatic reversal
Between 14 and 12 ka BP another climatic change known as the deglacial climatic reversal (DCR; Reference ThompsonThompson and others, 1998) was recorded in the Sajama ice core. The O18 values in ice from this period in the Sajama ice core are more depleted and approach LGS levels. This zone is broadly synchronous with other stadial events reported at Rıo Desaguadero, Bolivia (Reference Rigsby, Bradbury, Baker, Rollins and WarrenRigsby and others, 2005), in Lake Titicaca (Reference BakerBaker and others, 2001) and at Lake Compuerta on the western flank of the Peruvian Andes (Reference Weng, Bush, Curtis, Kolata, Dillehay and BinfordWeng and others, 2006). As reflected in the accumulation record at Sajama, this period corresponds to high stands in Lake Titicaca (Reference BakerBaker and others, 2001) and the Uyuni–Coipasa basin (Reference Sylvestre, Servant, Servant-Vildary, Causse, Fournier and YbertSylvestre and others, 1999; Reference Placzek, Quade and PatchettPlaczek and others, 2006) and a period of wet oscillations at Lake Pacucha (Reference Hillyer, Valencia, Bush, Silman and Steinitz-KannanHillyer and others, 2009).
Pollen concentrations in the DCR are close to values in the LGS and range from 130 to 262 grains L–1. The pollen percentages are dominated by Poaceae (40-74%), though high percentages of Asteraceae, Apiaceae, Plantago, Myrica, Polylepis, Caryophyllaceae, Cyperaceae and fern spores (Selaginella and Polypodicaeae-type) are present. The high percentages of Poaceae, along with the presence of Apiaceae and Cyperaceae, corroborate the wet conditions that were thought to exist on the Altiplano. Reference Kuentz, Galan de Mera, Ledru and ThouretKuentz and others (2007) found that during wet years, herbs (mainly Poaceae) may represent 75% of the vegetation cover in the orotropical vegetation zone. In addition to this, wetter conditions would expand the ‘bofedales’ or peat bog communities common to the Altiplano, where Poaceae, Apiaceae and Cyperaceae occur in abundance.
Holocene
After 12 kaBP, the chemical proxies from the ice core (Reference ThompsonThompson and others, 1998) indicate that the climate abruptly shifted to Holocene conditions and temperature and precipitation stabilized relatively quickly at ∼11 kaBP. Pollen concentrations in the core increase dramatically to 1500-1800 grains L–1, likely reflecting the warmer conditions on the Altiplano. The first notable amounts of charcoal appear at ∼12 kaBP and are found consistently throughout the Holocene, reinforcing the notion that fire has been an important ecological agent in the development of central Andean ecosystems (Reference BushBush and others, 2005). Though the Holocene transition is fairly abrupt, it is important to note that the pollen percentages for Poaceae and Asteraceae, the two dominant taxa, remained in a state of transition until 10 kaBP when their ratios stabilized near their modern-day levels.
Early Holocene (12-10kaBP) conditions are marked by an increase in percentages of Asteraceae pollen to an equilibrium level between 40 and 60%. Poaceae declines from its dominance in the late Pleistocene to an equilibrium level between 20 and 30%. We interpret this change as a response to a generally warmer and drier climate, which caused a contraction of the peat bog communities on the Altiplano and allowed the small shrubs that are present on the Altiplano today to replace the herbs common to the DCR. Increases in the Amaranthaceae families and decreases in the Apiaceae and Cyperaceae families support this interpretation (Reference GrafGraf, 1981).
In ice from 10.5 to 9.0 kaBP, pollen concentrations steadily increase from 1500 to 2250 grains L–1, then spike to their highest level in the record at 8500 (5000 grains L–1). We interpret this as a dry start to the Holocene with steadily increasing moisture levels that culminated with a wet event at ∼8.5 kaBP. In an ice cap there are two primary mechanisms that control pollen concentration: (1) pollen production/ influx, which is positively correlated to concentration; and (2) net accumulation, which is negatively correlated. As pollen production increases in the region, so does the amount of pollen that can potentially make it to the ice cap, thus increasing concentration. However, even if the pollen production remains constant in a given year, an increase in net accumulation will dilute the pollen, thereby reducing the pollen concentration. Fortunately, through most of the Holocene, the net accumulation rate on Sajama has been very constant (Reference ThompsonThompson and others, 1998). Because of this, the pollen concentration in the Holocene ice most likely reflects plant population density and pollen production. Many sources suggest that moisture is the critical (limiting) factor that determines plant population and pollen productivity on the Altiplano (e.g. Reference LuteynLuteyn, 1999); therefore, we interpret Holocene pollen concentration as a proxy for moisture availability. Also during this period (10.0-8.5 ka BP) Polylepis steadily increased, supporting the interpretation of decreasing moisture stress on Altiplano vegetation. Reference GrafGraf (1981) noted that increases in Polylepis pollen are not linked to drier conditions. It is important to note that the use of pollen as a moisture proxy should not be misinterpreted as a precipitation indicator, because many other factors (such as temperature, evaporation, cloudiness and wind conditions) contribute to the moisture availability for plants.
Other studies from the Andes show a similar climatic trend during the early Holocene. Low stands have been recorded at ∼10kaBP at Lake Junin (Reference Seltzer, Rodbell and BurnsSeltzer and others, 2000), Lake Titicaca (Reference Paduano, Bush, Baker, Fritz and SeltzerPaduano and others, 2003) and Lake Pacucha (Reference Hillyer, Valencia, Bush, Silman and Steinitz-KannanHillyer and others, 2009). Sediment profiles from these lakes also show increasing amounts of moisture after 10 ka BP, culminating in high stands at ∼8.5 ka BP (Reference BakerBaker and others, 2001; Reference Paduano, Bush, Baker, Fritz and SeltzerPaduano and others, 2003; Reference Hillyer, Valencia, Bush, Silman and Steinitz-KannanHillyer and others, 2009). Reference ThompsonThompson and others (1995) also note a similar moisture trend in the Huascaran ice core, though the wetter conditions there persisted until 7kaBP. Our findings are notably out of synch with the record from Laguna de Chochos, which shows a remarkable low stand between 9.5 and 7.3 ka BP (Reference BushBush and others, 2005).
In ice deposited after 8.5 kaBP, the pollen concentration drops markedly to 1260 grains L–1 and remains below 2000 grains L–1 until 5.5 kaBP. We interpret this period as corresponding to the mid-Holocene dry period that has been widely reported across the Andes (Reference Seltzer, Baker, Cross, Dunbar and FritzSeltzer and others, 1998; Reference BakerBaker and others, 2001; Reference Cross, Baker, Seltzer, Fritz and DunbarCross and others, 2001; Reference D’Agostino, Seltzer, Baker, Fritz and DunbarD’Agostino and others, 2002; Reference AbbottAbbott and others, 2003; Reference Paduano, Bush, Baker, Fritz and SeltzerPaduano and others, 2003; Reference Tapia, Fritz, Baker, Seltzer and DunbarTapia and others, 2003; Reference Bush, Silman and UrregoBush and others, 2004; Reference MorenoMoreno and others, 2007; Reference Ekdahl, Fritz, Baker, Rigsby and ColeyEkdahl and others, 2008; Reference Hillyer, Valencia, Bush, Silman and Steinitz-KannanHillyer and others, 2009; Reference Urrego, Bush and SilmanUrrego and others, 2010). This period also corresponds to an interval of marked retreat of Quelccaya ice cap, signaling not only drier but probably warmer conditions as well (Reference Buffen, Thompson, Mosley-Thompson and HuhBuffen and others, 2009). Several studies have suggested that El Nino was weakened or less active during this period (Reference Moy, Seltzer, Rodbell and AndersonMoy and others, 2002) and may have exacerbated these dry conditions. Aside from pollen concentration, the percentages of individual taxa do not respond significantly to this event. One notable exception is a sharp decline in Polylepis at ∼7kaBP. Toward the end of this dry event (6kaBP) there is a small increase in Cyperaceae and the first significant rise in charcoal. Reference Hillyer, Valencia, Bush, Silman and Steinitz-KannanHillyer and others (2009) found an increased frequency of short wet events or oscillations near the end of the mid-Holocene dry period, and the increase of charcoal and Cyperaceae may corroborate this.
In ice deposited after 5.5 kaBP, pollen concentration returns to its pre-dry-period level and maintains these high levels (2000-3500 grains L–1) to present day. During this period there are no marked changes in the percentages of individual pollen taxa, signaling relatively stable conditions. Remarkably, this trend holds true for Polylepis, which maintains percentages near 10% throughout the rest of the Holocene. Most pollen studies in the Andes show declining abundance of Polylepis (e.g. Reference Hansen and RodbellHansen and Rodbell, 1995; Reference Colinvaux, Bush, Steinitz-Kannan and MillerColinvaux and others, 1997; Reference BushBush and others, 2005) in the late Holocene, a trend thought to indicate widespread reductions in populations of Polylepis due to increased fire and other human disturbances (Reference Fjeldsa and KesslerFjeldsa and Kessler, 1996; Reference Cingolani, Renison, Tecco, Gurvich and CabidoCingolani and others, 2008). The consistency of our numbers through the late Holocene may reflect the close proximity of thin stands of Polylepis, which are still present near the base of Sajama today. Also of note is the dramatic increase in charcoal to nearly 230 000 particles L–1 in the most recent sample. This is undoubtedly the result of anthropogenic burning and ore-smelting (Reference BushBush and others, 2005; Reference Cooke, Abbott and WolfeCooke and others, 2008).
Conclusion
This study shows that pollen in tropical ice cores can be used as an indicator of vegetation response to glacial/interglacial climate change sequences. Pollen is direct evidence from vegetation and thus adds an important biological proxy to the existing paleoenvironmental record at Sajama. An example of this added contribution is our demonstration that the vegetation response during the DCR was quite different than that seen in the LGS. The ice-core record shows both the DCR and the LGS as cold and wet periods, with the Last Glacial Maximum (LGM) being wetter (Reference ThompsonThompson and others, 1998), yet the plants show quite a different response. It can be speculated that the whole area was largely snow-covered during the LGM, eliminating most of the regional vegetation. A large part of the snow cover was likely lost during the warm/dry interstadial such that Poaceae and Polylepis appear to have flourished during the cold and drier DCR. Other examples can be seen in the dominance of dry taxa (Asteraceae, Amaranthaceae) during the Bølling-Allerød/Guantiva interstadial, as well as the timing and duration of the vegetation transition into the Holocene.
It is important to note, however, that these pollen data should be interpreted with caution in areas of the core with low pollen concentration or in samples with an over-representation of a handful of taxa. For example, in many samples Asteraceae and Poaceae pollen account for >80% of the total pollen sum. Though these numbers reflect the overwhelming abundance of these plants on the Altiplano, this greatly limits the percentages of other pollen taxa, reducing their sensitivity as indicators of vegetation change. Another useful parameter in the pollen record is pollen concentration, which can be used as a moisture indicator when net accumulation is stable. At several important transitions (end of LGS, DCR, and start of the Holocene), pollen concentration responded in unison with the O1 8 and accumulation data from Sajama. However, the causes driving these changes in moisture availability cannot be detected with the pollen alone.
In general, the pollen data corroborate many of the paleoenvironmental changes recorded at Sajama and throughout the Andes. LGS conditions persisted until ∼15.5 kaBP, followed by a 1500year period of warmer conditions. Starting at 14kaBP there was a return to stadial conditions corresponding with the DCR. The beginning of the Holocene at ∼12 ka BP was probably dry, with increasing amounts of moisture until about 8.5 kaBP. Low pollen concentrations reflect a dry mid-Holocene from 8.0 to 5.5 kaBP, with wetter conditions then re-emerging and holding stable to the present.
One interesting observation is that the timing of the mid-Holocene dry period (based on the pollen evidence) is slightly out of synch with the spikes in dust and in moisture-sensitive soluble anions (SO4– and Cl–) from the Sajama ice core, which occur between 6 and 2 ka BP (Reference ThompsonThompson and others, 1998). Different pollen source regions could account for these differences. Reference Hillyer, Valencia, Bush, Silman and Steinitz-KannanHillyer and others (2009) suggested that the mid-Holocene dry event was time-transgressive, occurring earlier in the northern Altiplano and later in the southern Altiplano near Sajama. The pollen at Sajama could represent source regions further to the north and northeast. This would agree with the summer circulation pattern on the Altiplano and also explain the strong correlation between the pollen on Sajama and the Lake Titicaca and Lake Pacucha records (Reference Paduano, Bush, Baker, Fritz and SeltzerPaduano and others, 2003; Reference Hillyer, Valencia, Bush, Silman and Steinitz-KannanHillyer and others, 2009). Although our data have corroborated the mid-Holocene dry event from many paleoenvironmental records around the Andes, it is notably out of synch with records from Lago Chaka Kkota and Laguna Viscachani (Reference Abbott, Seltzer, Kelts and SouthonAbbott and others, 1997, Reference Abbott, Wolfe, Aravena, Wolfe and Seltzer2000, Reference Abbott2003) and Lake Aricota (Reference Placzek, Quade and BetancourtPlaczek and others, 2001), which are relatively close to Sajama; this asymmetry warrants further investigation. In conclusion, the ice-core pollen record at Sajama is sensitive to climatic changes at this temporal scale, adds depth to the existing Sajama ice-core record, and corroborates many of its findings (based on chemical and physical evidence) with a biological proxy.
Acknowledgements
We thank Mary Davis for ice-core sample preparation, and Mary Lee Eggart and Cliff Duplechin for cartographic assistance. We also thank Sally Horn and an anonymous reviewer for helpful suggestions. This research was jointly funded by the Geography and Regional Science Program, the Paleoclimate Program, and the Americas Program of the US National Science Foundation (NSF grants BCS-9906002, BCS-0117338 and BCS-0217321).