INTRODUCTION
The backbone of the outstanding abundance sensitivity of accelerator mass spectrometry (AMS) is its ability to nearly completely suppress isobaric interferences. Given a typical mass resolution of AMS spectrometers of M/ΔM ≈ 300, all ion species with the same integer mass as the isotope of interest require suppression in one of the multiple filters of an AMS instrument. Molecular isobars are completely dissociated in the stripping process, by selecting high charge states that lack the electrons for molecular binding or by collisional dissociation in a rather dense stripper medium (Schulze-König et al. Reference Schulze-König, Seiler, Suter, Wacker and Synal2011). Either way, most fragments are subsequently separated in the high energy spectrometer and only m/q ambiguities of the isotope of interest can reach the detection setup, albeit at a different, typically lower charge state and thus different kinetic energy (Kilius et al. Reference Kilius, Zhao, Litherland and Purser1997). Provided that their intensities do not overload the detection setup, they can be fully separated by total energy detection. For several AMS isotopes like 14C, 26Al, 55Fe or 129I, this is sufficient for a background-free identification of the isotope of interest as no anions of these atomic isobars exist.
The majority of long-lived radioisotopes, however, have at least one abundant stable atomic isobar with the ability to form negative ions. The capabilities of sample preparation chemistry in isobar reduction typically end at the 0.1–1 ppm level, thus detection levels of 10−12–10−15 are only achievably with instrumental isobar suppression by many orders of magnitude. While a favorable choice of the anion species from the ion source (hydrides, oxides, fluorides) may partly accomplish this (e.g., Raisbeck et al. Reference Raisbeck, Yiou, Peghaire, Guillot and Uzureau1981; Vockenhuber et al. Reference Vockenhuber, Bichler, Golser, Kutschera, Priller, Steier and Winkler2004), isobar suppression typically relies on nuclear physics means in the detection setup: differential energy loss, gas-filled-magnets, degrader or absorber foils or full stripping. All of these techniques have in common that their performance increases with kinetic energy or velocity of the ions and that they become less efficient the smaller the relative difference in atomic number between isotope of interest and isobar, i.e., for high Z-nuclides (for an overview, see Synal Reference Synal2013). While 10Be-10B can nowadays be sufficiently separated at 720 keV kinetic energy from a 300 kV tandem (Maxeiner et al. Reference Maxeiner, Synal, Christl, Suter, Müller and Vockenhuber2019), 60Fe-60Ni already requires >110 MeV from a >11 MV tandem (Ludwig et al. Reference Ludwig, Bishop, Egli, Chernenko, Deneva, Faestermann, Famulok, Fimiani, Gómez-Guzmán and Hain2016; Wallner et al. Reference Wallner, Feige, Kinoshita, Paul, Fifield, Golser, Honda, Linnemann, Matsuzaki and Merchel2016) and 81Kr-81Br could only be separated to the 81Kr/Kr level of 10−13 at 3.6 GeV kinetic energy from a cyclotron (Collon et al. Reference Collon, Kutschera, Loosli, Lehmann, Purtschert, Love, Sampson, Anthony, Cole and Davids2000). Given the vast part of the nuclear chart that cannot be accessed by these techniques (cf. Figure 2) and the progressing scarcity of large tandem facilities, alternative techniques for isobar suppression independent of attainable particle energy have come into focus. Ideally, they may enable measurement of a range of radionuclides at compact AMS facilities now dedicated to radiocarbon only or even dispensing the accelerator-A from AMS.
The idea to exploit differences in electron affinities (EA) for isobar suppression of anions by laser photodetachment has been explored from the early days of AMS (Berkovits et al. Reference Berkovits, Boaretto, Hollos, Kutschera, Naaman, Paul and Vager1989), however it took another 20 years of development to attain sufficient continuous isobar depletion by extending ion-laser interaction times to ms in a linear radiofrequency-quadrupole (RFQ)-ion guide (Liu et al. Reference Liu, Beene, Havener and Liang2005; Andersson et al. Reference Andersson, Lindahl, Hanstorp, Havener, Liu and Liu2010). In 2016, the first Ion-Laser InterAction Mass Spectrometry (ILIAMS) setup based on this principle was coupled to the state-of-the-art 3-MV-AMS facility VERA (Vienna Environmental Research Accelerator) (Steier et al. Reference Steier, Golser, Kutschera, Priller, Vockenhuber and Winkler2004; Golser et al. Reference Golser and Kutschera2017). After 5 years of successful operation, it’s time for a review of this technique, its achievements, and its potential.
The ILIAMS-Technique—Advantages and Challenges
The layout of the ILIAMS setup including a schematic of VERA is shown in Figure 1 and detailed descriptions are given in Martschini et al. (Reference Martschini, Pitters, Moreau, Andersson, Forstner, Hanstorp, Lachner, Liu, Priller, Steier and Golser2017, Reference Martschini, Hanstorp, Lachner, Marek, Priller, Steier, Wasserburger and Golser2019), the essentials are repeated briefly here. Negative ions from a Multicathode-SNICS ion source are accelerated to 30 keV energy and mass-analyzed with a 90° bending magnet. Next, the ion beam is electrostatically decelerated to 30–60 eV by a high voltage platform and enters a 1-m-long RFQ-ion guide mounted there. Being filled with buffer gas, typically He at pressures of 3–5 Pa, it serves as an ion cooler and further thermalizes the ion beam to kinetic energies lower than 1 eV. Inside the cooler, the ion beam is collinearly overlapped with an intense laser beam with a photon flux of ∼5 × 1020 cm−2s−1. Owing to ion-laser interaction times on the order of ms inside the cooler (Martschini et al. Reference Martschini, Pitters, Moreau, Andersson, Forstner, Hanstorp, Lachner, Liu, Priller, Steier and Golser2017), ion species with EAs below the photon energy are efficiently neutralized by photodetachment, i.e., >99.99%, despite low cross sections on the order of 10−17 cm2 for this non-resonant process. At the same time, anions with EAs higher than the photon energy stay unaffected. All surviving anions are reaccelerated to 30 keV upon leaving the high voltage platform, energy analyzed by an ESA and transported to the AMS instrument, where another mass filter needs to be passed before injection into the tandem. Since the degree of suppression does not depend on terminal voltage, the ILIAMS-setup can be coupled in principle to any AMS facility.
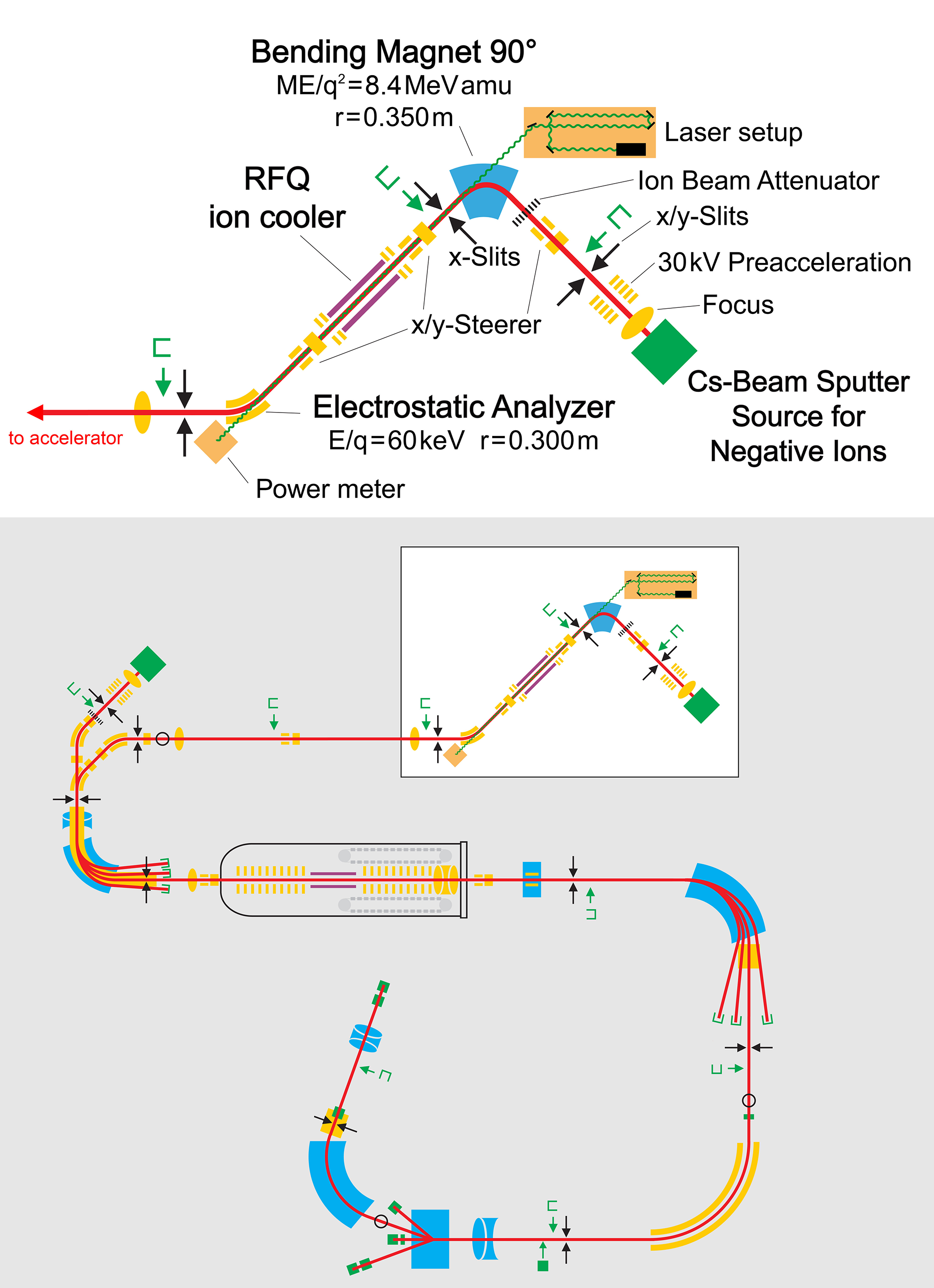
Figure 1 Layout of the ILIAMS setup and its coupling to the VERA AMS facility. Electrostatic components are shown in yellow, magnetic components in blue and components for ion generation and detection in green. (Please see electronic version for color figures.)
Differences in EAs or, in case of molecules, in vertical detachment energies (VDE) for isobar pairs are typically at least several tenths of eV (Andersen et al. Reference Andersen, Haugen and Hotop1999; Rienstra-Kiracofe et al. Reference Rienstra-Kiracofe, Tschumper, Schaefer, Nandi and Ellison2002) such that commercial lasers of suitable photon energy are usually readily available. Currently, these may either be cw-lasers or pulsed laser with repetition rates above several ten kHz. In case atomic anions have the wrong order of EAs for ILIAMS isobar suppression, a suitable molecular system has to be found to meet the requirements. The proper choice of molecular species sometimes requires tedious experimental work as only scarce data on the EAs or VDEs of prolific molecules like fluorides or oxides is available. In addition, a substantial fraction of molecular ions from a sputter ion source is heavily excited (Gnaser Reference Gnaser2007) and may be photodetached at energies substantially lower than their EA or VDE. Thus, EA/VDE differences in molecular situations should be as large as possible to mitigate losses of the anion of interest.
A complimentary technical approach termed Isobar Separator for Anions (ISA) exploits the very same differences in electronic structure for isobar suppression via resonant charge transfer reactions with reactive gases in a similar ion guide (Litherland et al. Reference Litherland, Tomski, Zhao, Cousins, Doupé, Javahery and Kieser2007). Demonstration experiments suggest suppression factors of 5–6 orders of magnitude (Eliades et al. Reference Eliades, Litherland, Kieser, Cousins, Ye and Zhao2010), although no results from full AMS measurements have been published so far. An advantage of the ISA technique is that changing gas is certainly cheaper and quicker than switching to yet another laser for a new isotope. However, ISA isobar suppression seems to be ultimately limited by a plateau effect (Eliades et al. Reference Eliades, Zhao, Litherland and Kieser2015). We observe similar behavior in our setup when running the system without lasers and using reactive gases instead of pure He to induce molecular reactions (e.g., WF5 −→ WF5O−) (Martschini et al. Reference Martschini, Lachner, Merchel, Priller, Steier, Wallner, Wieser and Golser2020). In our interpretation, this plateau effect is determined by the likelihood of reverse reactions when the chemical equilibrium inside the ion cooler is reached. While the stability criterion of an RFQ ion guide typically allows the extra electron to stay in the system when bound to the modified molecule or resonantly transferred to a gas molecule, a free electron detached by lasers has no stable trajectory in the RFQ and is immediately removed from the system to one of the electrodes. Thus, a reverse reaction of the photodetachment process is virtually impossible and no plateau effect has been observed with laser photodetachment, paving the way for isobar suppression factors of >1010, i.e., “complete” isobar suppression (see below).
A challenge in AMS context for ion guide techniques is the intensity of the ion beam as RFQ-ion guides typically cannot handle beam currents of several μA or above. In order not to hamper conventional AMS measurements, these ion guides should therefore be either removable or part of a separate injector. Of course, this current limitation also applies to the stable reference isotope during ILIAMS measurements. Even at ion currents of several 100 nA, space charge influences both the beam transmission as well as the ion residence time in the system (Martschini et al. Reference Martschini, Pitters, Moreau, Andersson, Forstner, Hanstorp, Lachner, Liu, Priller, Steier and Golser2017, Reference Martschini, Hanstorp, Lachner, Marek, Priller, Steier, Wasserburger and Golser2019). Consequently, stable reference isotope beams need to be attenuated in a reproducible way conserving as much as possible from the original phase space. At VERA, this is currently accomplished by slow sequential injection of isotopes and pneumatically inserting a perforated metal sheet, i.e., an industrial sieve, into the beam during stable isotope sequences. Typical sequence durations are 100–250 s counting on the rare isotope followed by 5–10 s of stable reference isotope injection. The reproducibility of AMS results with this method is around 3% (Lachner et al. Reference Lachner, Marek, Martschini, Priller, Steier and Golser2019, Reference Lachner, Martschini, Kalb, Kern, Marchhart, Plasser, Priller, Steier, Wieser and Golser2021), which is dominated by an observed inter-target scatter among results from multiple sputter targets of the same sample material rather than the statistical precision (cf. Table 1) or reproducibility on a single sputter target over multiple runs. We attribute this to variations in ion beam loss during injection into the cooler caused by differences in sample cratering and/or a slight eccentricity of the sample wheel. Nonetheless, the present reproducibility meets the requirements for most established AMS nuclides other than 14C and certainly is satisfactory for nuclides previously inaccessible at environmental levels.
Table 1 Key performance parameters of ILIAMS AMS at VERA for the 4 established isotopes. Values given are averages over various beamtimes in the past and not the best values observed so far.

(a) Unattenuated reference isotope current in front of the ion cooler, values for Cl and Sr are 35Cl and 88Sr resp.
(b) Nominal isotopic ratio divided by the raw measured isotopic ratio. Usually, values are above 1 due to limited ROI acceptance in the detection setup. Values below 1.0 indicate that the transmission of the rare isotope through the system, especially the ion cooler, is higher than for the reference isotope.
(c) Rare isotope counts collected within a 100 s long continuous counting sequence.
(d) Rare isotope counts collected within 10 min sputter time in measurement mode considering times lost for reference isotope injection, switching between setups for slow sequential injection, counting of other masses, etc.
STATUS AND PROSPECTS
In the following, the current experimental situation and prospects of the ILIAMS-technique for several successfully established AMS nuclides as well as some interesting new candidates will be discussed including requirements for their subsequent AMS detection. An overview of nuclides measured with ILIAMS is shown in Figure 2. Results presented below were obtained with one of the three following laser systems: 532 nm (2.33 eV photon energy, cw, VERDI V18, Coherent Inc.), 355 nm (3.49 eV, 100 kHz repetition rate, AVIA-LX, Coherent Inc.) 455 nm (2.72 eV, cw, Spyder Arctic III, Wicked Lasers). Individual events were counted in a split-anode gas ionization chamber (GIC) on the high energy side of VERA (Forstner et al. Reference Forstner, Michlmayr, Auer, Golser, Kutschera, Priller, Steier and Wallner2008).

Figure 2 Nuclear chart showing long-lived radionuclides of interest and potential interest for AMS. Nuclides are coded according to their present accessibility with AMS and the development status of ILIAMS. Half-lives in Myr are indicated in the round brackets.
Fully Developed Stage
ILIAMS is successfully applied in full AMS measurements of these isotopes at VERA, the respective key performance parameters are summarized in Table 1.
36 Cl
With its high EA of 3.6 eV, 36Cl is the prototype radionuclide for ILIAMS and is routinely measured with this technique at VERA for 4 years (Lachner et al. Reference Lachner, Marek, Martschini, Priller, Steier and Golser2019). A 532 nm laser with 10 W power can reduce the intensity of the isobar 36S (EA 2.1 eV) by more than 10 orders of magnitude at an overall 36Cl transmission through the ion guide of up to 80%. This essentially omits the need for any further atomic isobar discrimination and blank values below 36Cl/Cl = 10−15 become accessible at beam energies of only 5.4 MeV. Sulfur-spiked samples demonstrate that this background stems from 36Cl contamination in the ion source and during sample handling and not from isobaric interference, which is several orders of magnitude lower. Using the highly populated 2+ charge state in the HE-spectrometer after the VERA accelerator, no other ion species than 36Cl2+ is observed in the GIC. Before stripping in the accelerator, 17O19F− is typically the only isobaric ion species in the m=36-beam after ILIAMS, all other possible interferences like O2 − and C3 − are completely suppressed in the RFQ with > 2 W of laser power. Since the EA of 17O19F− is 2.27 eV (Rienstra-Kiracofe et al. Reference Rienstra-Kiracofe, Tschumper, Schaefer, Nandi and Ellison2002), photons with higher energy (e.g., from the 355 nm laser) are expected to sufficiently remove also this last interference, which could pave the way for accelerator-free detection of 36Cl.
26 Al
In principle, AMS of 26Al completely avoids atomic isobars when selecting the elemental anion Al− for injection because Mg has a negative EA (Andersen et al. Reference Andersen, Haugen and Hotop1999). On the other hand, the oxide anion AlO− typically yields 10 times higher ion currents and ionization efficiencies from an Al2O3 sputter matrix, at the cost of MgO− interference. Fortunately, ILIAMS provides unprecedented suppression of MgO− of >1014 at ∼50% overall transmission of AlO− through the ion guide and allows to exploit the benefits of higher overall detection efficiency (Lachner et al. Reference Lachner, Martschini, Kalb, Kern, Marchhart, Plasser, Priller, Steier, Wieser and Golser2021). Despite a published VDE of 1.61 eV (Cheng et al. Reference Cheng, Berkdemir, Melko and Castleman2013), MgO− is suppressed by up to 105 by simple passage through pure He and 16O− is found in the ion beam exiting the cooler. While clearly suggesting dissociation reactions, this behavior is still not fully understood and e.g., not observed for MnO−, a diatomic anion with similar EA (see below). Photons from the 532 nm laser “completely” remove any remaining MgO− with measured suppression factors of 1011. Hence, only m/q-ambiguity-fragments of other molecules may reach the GIC, most notably 13C1+ when selecting the 2+ charge state after the accelerator and may warrant use of the less populated 3+ charge state for samples with elevated C-content. Even the 1+ charge state could be an option at compact facilities provided that interference from molecular fragments of 10B16O2 −, 13C2 16O− and 13C12CH16O− can be suppressed either with ILIAMS or in the terminal stripper. The gain in overall detection efficiency with ILIAMS at VERA is 3–5, which substantially cuts down measurement times. Especially low-level samples benefit from the improved counting statistics and blanks are measured down to 26Al/27Al = 5×10–16. This method has become the standard technique for AMS of 26Al at VERA (Lachner et al. Reference Lachner, Martschini, Kalb, Kern, Marchhart, Plasser, Priller, Steier, Wieser and Golser2021).
90 Sr
The fission product 90Sr (T1/2 = 28.64 a) is of high environmental interest both for its radiotoxicity and its potential as a tracer, but conventional AMS detection is complicated by the presence of the isobar 90Zr and, to a lesser extent, 90Y. ILIAMS provides a suppression of ZrF3 – and YF3 – vs. SrF3 – of >107 with 12 W of power from the 532 nm laser and admixing 3% of O2 to the He buffer gas (Martschini et al. in preparation). Interestingly, the degree of suppression by photons or gas alone is 1–2 orders of magnitude lower, which means that the two effects obviously do not multiply. The exact reaction channels have not been studied so far but ISA results support the low stability of ZrF3 – (Eliades et al. Reference Eliades, Zhao, Litherland and Kieser2013, Reference Eliades, Zhao, Litherland and Kieser2015).
Extraction of SrF3 – from the ion source and elemental separation in the GIC at 10.85 MeV ion energy (3 MV, 3+ charge state) provide additional suppression of Zr of >105. The mean blank value from commercial SrF2 as well as full chemistry blanks from 90Sr-free soil is 90Sr/Sr = (4.5±3.2)×10–15 at an overall Sr-detection efficiency of 4×10–4 and results in a detection limit of 0.08 mBq. This corresponds to more than a 30-fold improvement of previous AMS benchmarks, which were similar to the radiometric limit of 3 mBq (Tumey et al. Reference Tumey, Brown, Hamilton and Hillegonds2009; Sasa et al. Reference Sasa, Honda, Hosoya, Takahashi, Takano, Ochiai, Sakaguchi, Kurita, Satou and Sueki2021). Studies of 90Sr in environmental samples with ILIAMS have been initiated.
135,137 Cs
The radioisotopes 135Cs (T1/2 = 2×106 a) and 137Cs (T1/2 = 30.17 a) are produced with high yield in nuclear fission with the latter being a well-established environmental tracer. Gaining access to the isotopic ratio 135Cs/137Cs would have significant advantage for source identification but AMS detection attempts were rather scarce, both due to isobaric interference from stable Ba-isotopes as well as the challenge posed by the use of Cs in typical AMS sputter ion sources. ISA experiments tackled the first problem and identified CsF2 – as a suitable anion species for ion guide techniques (Eliades et al. Reference Eliades, Zhao, Litherland and Kieser2013; MacDonald et al. Reference MacDonald, Charles, Cornett, Zhao, Kieser and Litherland2014).
ILIAMS enables the suppression of the isobar BaF2 – with the 532 nm laser by a factor 105 against CsF2 – at more than 30% transmission of the CsF2 –-beam from the ion source through the ILIAMS setup. After the accelerator, the most populated charge state 3+ is chosen with a stripping yield of ∼23%. Anyway, the GIC does not contribute to the elemental discrimination from Ba and even at a beam energy of 27 MeV (achieved by selecting the sparsely populated 8+ charge state), Ba ends up in the same spot of the GIC-spectra as Cs. Consequently, the Ba-contribution to the respective Cs-signals has to be inferred from monitoring the count rate of 136Ba.
In the ion source, Rb is used as sputter material and yields CsF2 –-beam currents of up to 100 nA from a Cs2SO4+PbF2+Cu sputter matrix. Typically, BaF2 – is fully suppressed and only Ba-spiked samples show some 136Ba3+-events. Blank values measured on commercial Cs2SO4 presently are 137Cs/133Cs = 3×10–12 and 135Cs/133Cs = 6×10–12 and represent new AMS benchmarks. Since 137Ba is more abundant compared to 135Ba, these values suggest that the detection limit of 135Cs is either determined by the intrinsic 135Cs content of commercial Cs-materials, by cross contamination in the ion source or by some yet unidentified interference at mass 135. Nonetheless, studies of environmental 135Cs-levels are now feasible and being conducted.
In-Depth Investigation Stage
These isotopes have been investigated with ILIAMS, but further research work is required before full AMS measurements with environmental samples are feasible.
41 Ca
AMS of 41Ca (T1/2 = 1.03×105 a) for both cosmogenic and medical applications suffers from interference of 41K. The ion species of choice is CaF3 − due to the hassle involved in chemical preparation and handling of CaH2 despite better AMS performance of the latter (Wallner et al. Reference Wallner, Forstner, Golser, Korschinek, Kutschera, Priller, Steier and Vockenhuber2010). ISA-results demonstrated that suppression of KF3 − vs. CaF3 − is possible inside ion guides (Zhao et al. Reference Zhao, Litherland, Eliades, Fu and Kieser2016), despite that KF3 − is a superhalogen anion with a calculated VDE of 6.83 eV (Lo and Hopkinson Reference Lo and Hopkinson2011). The breakup into KF2 − and F was identified as the main reaction channel with a theoretical threshold energy of 1.0 eV.
With ILIAMS, less than a factor of 10 suppression of KF3 −is observed at 532 nm, while 10 W of 355 nm laser yield suppression factors of up to 104 at no laser-induced loss of 41Ca. Provided that the calculated VDE of KF3 − is correct within a factor of 2, this implies that photodetachment of the electron is energetically not possible and the observed removal of KF3 − can only stem from dissociation processes. Calculated bond dissociation energies for the reaction channels KF3 − → KF2 − + F, KF3 − → KF2 + F− and KF3 − → KF + F2 − are all below 2.3 eV (Lo and Hopkinson Reference Lo and Hopkinson2011). Which of these reaction pathways plays the dominant role and why it only becomes strongly enhanced in the presence of 3.49 eV photons but not with 2.33 eV photons remains to be investigated.
Sample spectra obtained on K-spiked blank material as well as SMD-Ca-10 reference material (Rugel et al. Reference Rugel, Pavetich, Akhmadaliev, Enamorado Baez, Scharf, Ziegenrücker and Merchel2016) are shown in Figure 3. These were acquired by selecting the 4+ charge state after the accelerator with a charge state yield at 3 MV of 11% providing enough energy for further isobar suppression in the GIC. While there are still single 41K events visible from the K-spiked sample, the use of the 3+ charge state with a yield of 22% or even lower charge states seems feasible and will be explored in the near future. This may partially compensate the present ion optical loss of ∼85% of CaF3 − during injection into the ion guide, which is a consequence of the poor beam emittance from the source caused by the presence of > 50 μA F−-current. Similar problems occur for most fluoride anions, but the ratio of F− to the species of interest is exceptionally high in the case of CaF3 −. Whether the observed KF3 −-suppression can be enhanced to an extent that no subsequent isobar discrimination in the detector is required for environmental Ca samples remains open. The detection limit of 41Ca/Ca in the low 10−14 is currently set by the low overall detection efficiency rather than isobaric background.
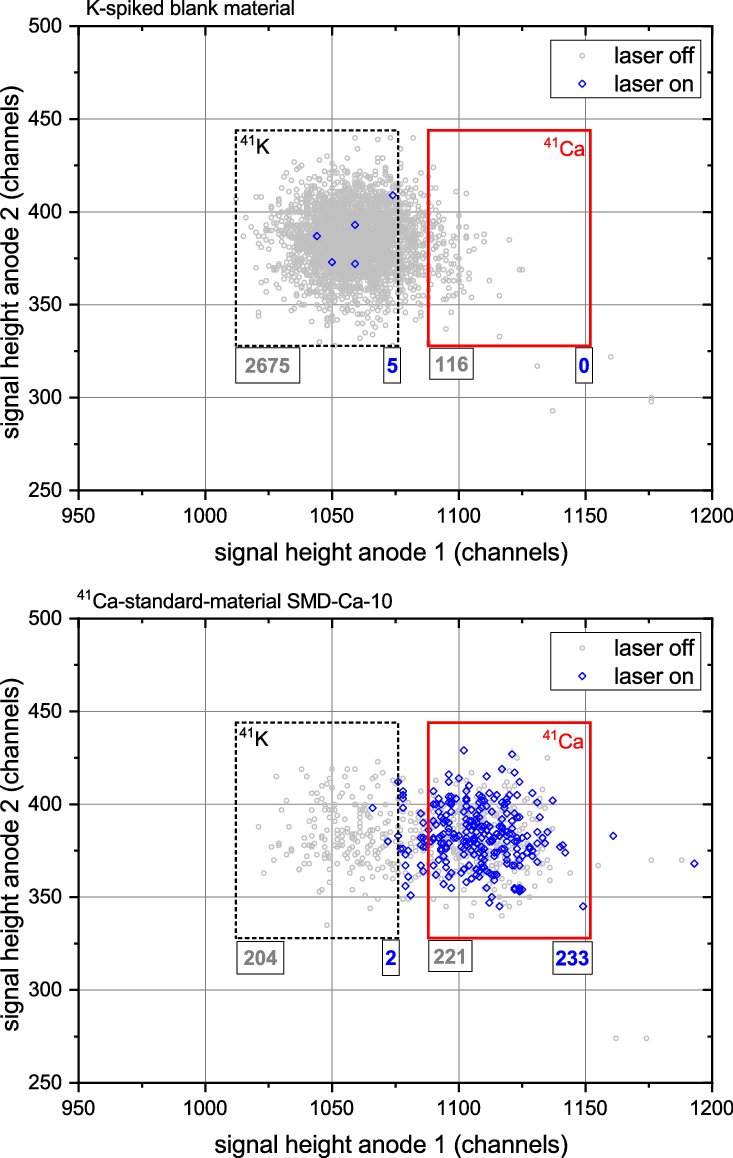
Figure 3 Sample spectra of 41Ca4+ and 41K4+ acquired at 13.26 MeV during an ILIAMS-beamtime. Gray events were collected without laser, events indicated as blue diamonds were collected with 1.5 W of 355 nm laser. The upper graph shows the energy loss spectra from commercial CaF2 material spiked with KNO3, the lower graph was collected on the reference material SMD-Ca-10 with a nominal 41Ca/40Ca ratio of (0.9985 ± 0.0054)×10−10 (Rugel et al. Reference Rugel, Pavetich, Akhmadaliev, Enamorado Baez, Scharf, Ziegenrücker and Merchel2016). Regions of interest for 41Ca and 41K are shown as rectangles; to the lower left corner the respective number of events within this ROI with laser off is given, and to the lower right, the number of events with laser on. Acquisition time was 100 s for all spectra. Despite running at reduced power, the laser light efficiently removes 41K while leaving 41Ca intact.
99 Tc
Only two of the largest AMS facilities have so far succeeded in separating 99Tc (T1/2 = 2.1×105 a) from its abundant isobar 99Ru to an extent that allows 99Tc-detection at levels of 106–108 atoms per sample (Wacker et al. Reference Wacker, Fifield and Tims2004; Koll et al. Reference Koll, Busser, Faestermann, Gómez-Guzmán, Hain, Kinast, Korschinek, Krieg, Lebert, Ludwig and Quinto2019). Since no stable isotope is available, AMS results either have to be normalized to another reference element, which bears the uncertainty of different negative ion yields and, in our case, ion guide transmissions and thus reduces precision to ∼30% when using TcO−, or each sample has to be spiked with known amounts of another Tc-isotope with reasonably long half-life, e.g., 97Tc. In the latter case, also Mo has to be suppressed as an additional isobar, which has hampered feasibility of this method so far.
ILIAMS development work for 99Tc is tedious, as no measurable ion currents of this element are available, the VDEs of most molecular species are unknown and the atomic anions of the isobars have higher EAs than Tc (Andersen et al. Reference Andersen, Haugen and Hotop1999). At VERA, TcF5 − has been experimentally identified as the most suitable anion exhibiting only ∼5% loss at 10 W of 532 nm laser light, while RuF5 − is reduced by up to a factor 105. This is surprising given a published EA of RuF5 − of 5.2 eV (Kuznetsov et al. Reference Kuznetsov, Korobov and Sidorov1989) and may indicate reaction pathways other than direct photodetachment of the surplus electron. Another advantage of this ion species is the intrinsic strong suppression of RuF5 − observed when sputtering these ions from a PbF2-matrix enriched with Nb or Fe powder (Cornett et al. Reference Cornett, Zhao, Hou and Kieser2019). Preliminary results from a dilution series suggest that the 99Ru-induced background with ILIAMS results in a 99Tc-detection limit of < 106 atoms for environmental samples. In the GIC, no discrimination between isobars is possible in the 3+ or 4+ charge state, and the 99Ru signal has to be inferred from the count rate of 101Ru.
However, the problem of proper normalization remains unresolved as the suppression of MoF5 − vs. TcF5 − has proven more difficult. At 455 nm, MoF5 − is suppressed by < 2 without observed effect on TcF5 − while the 355 nm laser already induces more than 90% loss of TcF5 − and suppresses MoF5 − by (only) 100. This is insufficient for practical use as Mo is ubiquitous and the presently required amount of 97Tc spike of >1013 atoms per sample is not practical, because of production limitations, detector count rate issues and last but not least sample contamination risks caused by low but present levels of 99Tc in the spike. Once a suitable way of normalizing the measured 99Tc count rates is found, AMS of 99Tc with ILIAMS should be straightforward independent of final ion energy.
182 Hf
Attempts to measure 182Hf of astrophysical origin at environmental concentrations with VERA have started early on but were hindered by isobaric background from 182W (Vockenhuber et al. Reference Vockenhuber, Bichler, Golser, Kutschera, Priller, Steier and Winkler2004; Forstner et al. Reference Forstner, Gnaser, Golser, Hanstorp, Martschini, Priller, Rohlén, Steier, Vockenhuber and Wallner2011). The status of 182Hf AMS with ILIAMS has been recently summarized in (Martschini et al. Reference Martschini, Lachner, Merchel, Priller, Steier, Wallner, Wieser and Golser2020). Both HfF5 − and its isobaric species WF5 − are strongly bound anions and none of our lasers could induce any suppression. Experimental data suggest that UV lasers around 266 nm would be necessary (Leopold et al. Reference Leopold, Rohlén, Andersson, Diehl, Eklund, Forstner, Hanstorp, Hultgren, Klason, Lindahl and Wendt2014) and we envision this step in the future being aware that these high photon energies might cause unfavorable intense electron release from ion guide materials. However, we have succeeded in using our ion guide as a reaction cell by admixing 3% O2 to the He buffer gas, which results in 105 suppression of WF5 − at ∼25% loss of HfF5 − due to collisional detachment. This method had been suggested by (Zhao et al. Reference Zhao, Eliades, Litherland, Kieser, Cornett and Charles2013) and we have identified the adduct reaction WF5 −→ WF5O− as the main reaction channel (Martschini et al. Reference Martschini, Hanstorp, Lachner, Marek, Priller, Steier, Wasserburger and Golser2019). Following correction of the W contribution to the 182-signal by monitoring the 183W count rate, blank values on commercial HfF4 (embedded in PbF2 matrix) of 182Hf/180Hf of (3.4±2.1)×10−14 are achieved. The GIC provides no additional isobar separation with 3+-ions such that similar detection limits should be within reach in lower charge states as well, provided that molecular background can be fully suppressed in the stripper medium.
Early Exploration Stage
With the following nuclides, only first tests have been conducted, mainly to identify suitable molecular anion species for ILIAMS. They will be discussed only briefly here but illustrate the huge potential of the method and hopefully bear good news in the near future.
32 Si
In order to enable AMS detection of 32Si other than at the largest tandem facilities (Morgenstern et al. Reference Morgenstern, Fifield, Tims and Ditchburn2010), several oxide molecules of Si have been investigated with ILIAMS regarding their potential for suppression of the ubiquitous isobar 32S. Atomic EAs do not permit separation (Andersen et al. Reference Andersen, Haugen and Hotop1999) and the cross sections for suppression of SiO−, both by collisions as well as photodetachment, were found to be larger than for its isobar SO−. Thus, only SiO2 − and SiO3 − can be considered as viable options. While the 532 nm laser destroys both SiO2 − and SO2 −, SiO3 − experiences no losses compared to a factor 2–4 reduction in transmitted SO3 − intensity. Higher photon energies may thus enhance separation of trioxides, unfortunately the 455 nm laser was not yet available during this study. At 355 nm, all of the above anions are detached.
53 Mn
Being of astrophysical and geological interest, 53Mn is another entry on the “large-tandems-only-list” as ample kinetic energy is required in AMS to separate the isobar 53Cr in a gas-filled-magnet (Gladkis et al. Reference Gladkis, Fifield, Morton, Barrows and Tims2007; Poutivtsev et al. Reference Poutivtsev, Dillmann, Faestermann, Knie, Korschinek, Lachner, Meier, Rugel and Wallner2010). Based on published EA data of Mn and Cr (Gutsev et al. Reference Gutsev, Rao, Jena, Li and Wang2000; Gutsev et al. Reference Gutsev, Jena, Zhai and Wang2001), the only anion species, which has reasonable ion yields from a MnO2 sputter matrix and is suited for ILIAMS, is MnO−, with an EA of 1.38 eV compared to 1.22 eV for CrO−. In a preliminary experiment, the transmission of MnO− through the ion cooler was 38% and no reactions with pure He-gas or admixture of O2 were observed. CrO− showed similar behavior. Based on the EA values, a laser with a wavelength between 905 nm and 1017 nm will be required.
60 Fe
AMS separation of the astrophysically relevant nuclide 60Fe from its isobar 60Ni is the picture book application of gas-filled magnets at large tandem facilities with detection limits of 60Fe/Fe below 10−16 (Ludwig et al. Reference Ludwig, Bishop, Egli, Chernenko, Deneva, Faestermann, Famulok, Fimiani, Gómez-Guzmán and Hain2016; Wallner et al. Reference Wallner, Feige, Kinoshita, Paul, Fifield, Golser, Honda, Linnemann, Matsuzaki and Merchel2016). Presently, ILIAMS cannot challenge these limits, as anion species combining proper EAs with high negative ion yield from a sputter source and good ion guide transmission have not been found yet. FeO− and NiO− differ only by 0.02 eV in EA (Rienstra-Kiracofe et al. Reference Rienstra-Kiracofe, Tschumper, Schaefer, Nandi and Ellison2002), fluorides and hydrides generally suffer from low ion yields and strong parasitic currents from the ion source hampering ion guide transmissions. None of the molecular anions studied with ILIAMS so far allows the generation of intense ion beams for the AMS system. As soon as total detection efficiency is limiting the sensitivity, additional isobar suppression at AMS facilities with 6–8 MV is no solution. While this may not sound optimistic, the study is not yet completed and molecular systems still not crossed from the short list are FeH−, FeF3 − and FeF4 −.
107 Pd
AMS of 107Pd once was briefly attempted with a 14 MV-tandem but revealed insufficient suppression of the strong interference from the isobar 107Ag (Korschinek et al. Reference Korschinek, Faestermann, Kastel, Knie, Maier, Fernandez-Niello, Rothenberger and Zerle1994). ILIAMS studies have identified PdF− as a likely suitable anion and possibly also PdO− and ruled out PdO2 −, which was found less stable than AgO2 −. With 10 W of 532 nm laser light, PdO−, AgO− and AgF− are all depleted by >99.9% while PdF− is only reduced by a factor of 3, nourishing the expectation that this wavelength is close to the detachment threshold of PdF− but well above that of AgF−. Further photodetachment studies with tunable lasers to find the optimum wavelength have been initiated.
CONCLUSIONS AND OUTLOOK
Over the past 5 years, ILIAMS has become a well-verified technique for isobar suppression and has significantly broadened the range of radionuclides accessible with medium-sized AMS facilities. It is now routinely applied for several isotopes at VERA and has been demonstrated to meet the requirements of AMS regarding reliability and robustness. One of the strengths of the method is its compatibility with, in principle, any subsequent AMS system; thus both compact facilities but also those with tandems >5 MV will profit. At least two other AMS facilities are currently developing new ILIAMS-setups and hopefully will soon contribute to this exciting field. The list of interesting candidates to explore with ILIAMS is long (cf. Figure 2). Once accessible at environmental levels, many of these isotopes will bring interesting new applications as was the case for e.g., 236U. Whether isobar separation with ILIAMS in this mass region is feasible, e.g., to measure 236Pu and 236Np in the presence of 236U, will be explored within the scope of a recently awarded grant.
ACKNOWLEDGMENTS
Part of this work was funded by the RADIATE project under the Grant Agreement 824096 from the EU Research and Innovation program HORIZON 2020 and by grants Austrian Science Fund (FWF): P 22164-N20 and Austrian Science Fund (FWF): P 31614-N28. The authors acknowledge financial support of the ILIAMS research activities by “Investitionsprojekte” of the University of Vienna. We thank all students and technical staff involved, without their dedication ILIAMS would not have come beyond the planning stage. As physicists we feel indebted to Maki Honda from the Japan Atomic Energy Agency, Silke Merchel from HZDR Dresden and Francesca Quinto from KIT Karlsruhe for their continuous advice and effort in developing the sample preparation procedures required for new AMS isotopes. We are grateful to Dag Hanstorp and his team at Gothenburg University for fruitful collaboration and laser advice. The VERA-team thanks all users and colleagues, who agreed to provide samples for this work.