1. Introduction
Lawsonite blueschists and eclogites are expected to be the prevailing lithotypes developing during deep subduction of the oceanic crust and should therefore be quite abundant in exhumed subduction complexes (Ravna et al. Reference Ravna, Andersen, Jolivet and De Capitani2010). Thermal and chemical models predict that lawsonite should be widespread in subducted oceanic crust at pressure higher than 1.5 GPa (Zack et al. Reference Zack, Rivers, Brumm and Kronz2004). Nevertheless, lawsonite-bearing eclogites have been described from only a few localities in the world (e.g. McBirney, Aoki & Bass, Reference McBirney, Aoki and Bass1967; Watson & Morton, Reference Watson and Morton1969; Krogh, Reference Krogh1982; Caron & Péquignot, Reference Caron and Péquignot1986; Lardeaux et al. Reference Lardeaux, Caron, Nisio, Péquignot and Boudeulle1986; Oh, Liou & Maruyama, Reference Oh, Liou and Maruyama1991; Ghent, Stout & Erdmer, Reference Ghent, Stout and Erdmer1993; Shibakusa & Maekawa, Reference Shibakusa and Maekawa1997; Parkinson et al. Reference Parkinson, Miyazaki, Wakita, Barber and Carswell1998; Carswell et al. Reference Carswell, Tucker, O'Brien and Krogh2003; Och et al. Reference Och, Leitch, Caprarelli and Watanabe2003; Usui et al. Reference Usui, Nakamura, Kobayashi and Maruyama2003; Altherr et al. Reference Altherr, Topuz, Marschall, Zack and Ludwing2004; Harlow et al. Reference Harlow, Hemming, Lallemant, Sisson and Soresen2004; Mattinson et al. Reference Mattinson, Zhang, Tsujimori and Liou2004; Tsujimori, Liou & Coleman, Reference Tsujimori, Liou and Coleman2005; Tsujimori et al. Reference Tsujimori, Sisson, Liou, Harlow and Sorensen2006a ,b; Davis & Whitney, Reference Davis and Whitney2006; Usui, Nakamura & Helmstaed, Reference Usui, Nakamura and Helmstaed2006; Zhang & Meng, Reference Zhang and Meng2006; Zhang, Meng & Wan, Reference Zhang, Meng and Wan2007; Ghent, Tinkhan & Marr, Reference Ghent, Tinkhan and Marr2009; Ravna et al. Reference Ravna, Andersen, Jolivet and De Capitani2010; Tsujimori & Ernst, Reference Tsujimori and Ernst2014; Vitale Brovarone & Beyssac, Reference Vitale Brovarone and Beyssac2014). When formed, lawsonite-bearing assemblages are observed to be highly unstable and their preservation requires to be accompained by substantial cooling and a rapid uplift (Compagnoni & Maffeo, Reference Compagnoni and Maffeo1973; Carswell, Reference Carswell1990; Poli & Schmidt, Reference Poli and Schmidt1995; Maruyama et al. Reference Maruyama, Isozaki, Kimura and Terabayashi1997; Okamoto & Maruyama, Reference Okamoto and Maruyama1999; Forneris & Holloway, Reference Forneris and Holloway2004; Zack et al. Reference Zack, Rivers, Brumm and Kronz2004; Zhang & Meng, Reference Zhang and Meng2006; Brun & Faccenna, Reference Brun and Faccenna2008; Agard et al. Reference Agard, Amato, Jolivet and Burov2009; Ravna et al. Reference Ravna, Andersen, Jolivet and De Capitani2010; López-Carmona et al. Reference López-Carmona, Kusky, Santhos and Abati2011; Zucali & Spalla, Reference Zucali and Spalla2011). Overprinting by heating and fluid infiltration can destroy mineral assemblages typical of blueschist- and eclogite-facies conditions; as a result these assemblages are rare, and those preserving lawsonite-bearing eclogites are the rarest of all (Davis & Whitney, Reference Davis and Whitney2006).
In this paper we present the structural and petrological study of a lawsonite-bearing eclogitic metagabbro, cropping out in the north-western sector of the metaophiolitic Voltri Massif (Ligurian Western Alps, Italy).
The main metagabbro body is a 20 m sized lens, in contact with Na-amphibole-bearing metasediments; both are interlayered with serpentinite schists. The area is characterized also by the occurrence of ophicalcites and serpentinites with a variable degree of carbonation, up to their complete transformation into listvenites. The latter are the result of hydrothermal metasomatic processes related to intense CO2-rich fluid circulation (Hansen et al. Reference Hansen, Dipple, Gordon and Kellet2005).
In the last few years both the occurrence of high-pressure C-bearing lithologies and the mechanisms of interaction of CO2-rich fluids with rocks have been extensively examined to evaluate the carbon fluxes in subduction zones and the turnover rate of CO2 to the atmosphere (e.g. Kerrick & Connolly, Reference Kerrick and Connolly1998; Ague & Nicolescu, Reference Ague and Nicolescu2014; Kelemen & Manning, Reference Kelemen and Manning2015).
The aim of this paper is dual: (i) to study the structural and petrological features of the lawsonite-bearing eclogitic metagabbro, to infer its P–T–Deformation history, and (ii) to derive further information on the tectono-metamorphic history of the area, i.e. to understand the tectonic evolution at a subduction plate interface (Cannaò et al. Reference Cannaò, Scambelluri, Agostini, Tonarini and Godard2016).
2. Geological background
The Voltri Massif is located at the southernmost termination of the Western Alps (Fig. 1a) and it is made up of metaophiolitic rocks associated with metasediments and slices of subcontinental lithospheric mantle. These rocks underwent a complex Alpine tectono-metamorphic evolution, with blueschist- to eclogite-facies peak metamorphism with variable retrogressive overprints (Chiesa et al. Reference Chiesa, Cortesogno, Forcella, Galli, Messiga, Pasquare, Pedemonte, Piccardo and Rossi1975; Capponi, Reference Capponi1987, Reference Capponi1991; Capponi et al. Reference Capponi, Gosso, Scambelluri, Siletto and Tallone1994; L. Crispini, unpub. Ph.D. thesis, Univ. degli Studi di Genova, 1996; Capponi & Crispini, Reference Capponi and Crispini2002, Reference Capponi and Crispini2008 b).

Figure 1. (a) Structural sketch map of the eastern Ligurian Alps and adjoining units; in the top left inset a structural sketch map of the Western Alps is shown. (b) Geological map of the studied area surrounding the Roboaro River.
The Voltri Massif metaophiolites largely consist of serpentinites with metagabbros and lenses of metabasite; metasediments represent the cover of the ophiolitic basement and encompass the whole range from quartzschist to calcschist and micaschist, and are locally associated with metabasites (Chiesa et al. Reference Chiesa, Cortesogno, Forcella, Galli, Messiga, Pasquare, Pedemonte, Piccardo and Rossi1975; Cortesogno & Forcella, Reference Cortesogno and Forcella1978). In the study area, slices of continent-derived metasediment (i.e. quartzites and marbles) and subcontinental mantle (i.e. lherzolites, harzburgites with minor pyroxenites and dunite bodies) occur. Outcrops of upper Eocene – lower Miocene sedimentary deposits of the Tertiary Piedmontese Basin (TPB) cover the Voltri Massif rocks in nonconformity.
In general, the main lithologies of the Voltri Massif show several superposed structures, achieved under different tectonic conditions and ranging from ductile to brittle regime (Capponi & Crispini, Reference Capponi and Crispini2002). The oldest structures are eclogite/blueschist-facies foliation and rootless hinges of isoclinal folds that lack continuity across outcrops; they are related to subduction events. Later D1/D2 similar folds form under Na-amphibole greenschist to greenschist sensu stricto metamorphic conditions; overprinting of D2 on the D1 folds caused type 3 and rarely type 2 (Ramsay & Huber, Reference Ramsay and Huber1987) interference patterns. The D1 and D2 schistosity produce a composite fabric (CF), which is the most evident surface on the field and controls the contacts between different lithologies.
Ductile structures recording extension parallel to the foliation (i.e. shear bands, boudins, foliation boudinage and extensional veins; Capponi & Crispini, Reference Capponi and Crispini1997) developed under greenschist-facies conditions.
A detailed description of the main structural features and evolution of the Voltri Massif can be found in Capponi (Reference Capponi1987, Reference Capponi1991), Capponi et al. (Reference Capponi, Gosso, Scambelluri, Siletto and Tallone1994), L. Crispini (unpub. Ph.D. thesis, Univ. degli Studi di Genova, 1996) and Capponi & Crispini (Reference Capponi and Crispini2002, Reference Capponi and Crispini2008 b).
3. Geology of the studied area
The study area is located along the Roboaro River (GPS coordinates N44.544686°, E8.436038°), near Sassello village. This area is part of the metaophiolitic Voltri Massif and is characterized by the occurrence of rocks with different paleogeographic origins (oceanic versus continental). The main lithologies are, in decreasing order of areal extent, serpentinite, metagabbro, metabasite lenses (15 to 20m wide) and ophicarbonate (Fig. 1b), derived from the ancient ocean-floor of the Ligurian Tethys; restricted outcrops of both ocean- (i.e. glaucophane-bearing calcschists) and continent-derived metasediments (i.e. dolostone) are present in the area; TPB sedimentary deposits widely occur.
We focused on an eclogitic metagabbro lens wrapped by serpentinites and glaucophanic metasediments. The serpentinites are antigorite-bearing with a mylonitic texture characterized by a pervasive foliation that dips NE–NW (Fig. 1b). They are affected by a variable degree of carbonation, propagating in mesh fractures (Fig. 2a) or in fractures parallel to the schistosity; locally serpentinites are completely transformed into listvenite.

Figure 2. (a) Brecciated and carbonated serpentinites. (b) Overview of the metagabbro body and the glaucophanic metasediment lens. (c) Isoclinal fold (D2) inside the metagabbro body deforming a Na-amphibole-rich blue layer. (d) Detail of the layering in the metagabbro body and boudinage of the Ca–Na-pyroxene-rich green layer. Pen for scale is 15 cm long; coin is 2.5 cm diameter.
The glaucophane-bearing metasediments are commonly associated with the metagabbro lenses (Fig. 2b); they include syn-kinematic stable calcite + dolomite + white mica + chlorite + Na-amphibole + titanite (replacing rutile) + quartz + opaque minerals (e.g. pyrite) and rare apatite; relict intrafoliar folds develop a pervasive axial plane schistosity moderately dipping to the NE–NW (Fig. 3).

Figure 3. Geological map of the Roboaro River area; the Schmidt nets (lower hemisphere) show the attitude of the composite fabric in different lithotypes.
The main schistosity in both the serpentinite and metasediment is a composite foliation, related to the superposition of synmetamorphic deformational events.
The metagabbro lens shows a peculiar alternation of Na-amphibole-rich blue layers and Ca–Na-pyroxene-rich green layers, with a mylonitic texture (Fig. 4). Both layers include isoclinal intrafoliar folds (D1) that are the oldest recognizable event. D1 folds develop a pervasive Na-amphibole-bearing axial plane schistosity. The metagabbro was affected by a following folding event (D2), testified to by isoclinal folds (Figs 2c, 3), deforming all the previous structures and causing the alternation of the green and blue bands; the superposition of D1 and D2 structures produced a composite fabric dipping NE–NW, which shows the same attitude of the surrounding serpentinite and metasediment schistosity. Extension of fold limbs caused a millimetre- to centimetre-scale dominant symmetric boudinage of the ‘rigid’ Ca–Na-pyroxene-rich green layers (Figs 2d, 4) and the development of low-angle shearing surfaces, defining an asymmetric boudinage, in the ‘soft’ Na-amphibole-rich blue layers. Indeed, the centimetre-thick Ca–Na-pyroxene-rich layers show prevailing straight to locally oblique necks, in which albite + titanite + Fe-oxide grow along the direction of maximum extension.

Figure 4. High-resolution scan of a sample from the metagabbro body, showing the peculiar green and blue layering; boudinage of the Ca–Na-pyroxene green layers and low-angle shearing surfaces in Na-amphibole-rich blue layers are visible.
The TPB outcrops are at the top of the meta-ophiolitic sequence and show a sub-horizontal bedding.
The study area is also characterized by two main fault-systems: one is represented by high-angle to sub-vertical normal faults, whereas the other system is represented by a low-angle thrust fault, with splays isolating sigmoidal bodies.
4. Petrography of the lawsonite-bearing eclogitic metagabbro
The alternating green and blue layers, defining the composite fabric of the metagabbro body, are Ca–Na-pyroxene and Na-amphibole-rich respectively.
The green layers include millimetre- to centimetre-sized porphyroclasts of magmatic Ca-pyroxene (augite) that hosts sulphurs and that are partially to totally replaced by coronitic Ca–Na-pyroxene (1; omphacite composition; Fig. 5a, b); fringes of Na-amphibole + pyrite or titanite grow from Ca–Na-pyroxene crystals (1), gradually replacing them (Fig. 5b–f). Ca–Na-pyroxene (2) recrystallizes as syn-kinematic subgrains (Fig. 5a, c, d, e). Rare garnet includes fine-grained Na-amphibole (1) in the core (Fig. 6a) and grows in equilibrium with Ca–Na-pyroxene. Lawsonite nucleates in the Na-amphibole (2) pressure shadows growing from Ca–Na-pyroxene (1) and is replaced by later albite (Figs 5f, 6b).

Figure 5. Photomicrographs of representative minerals and structures in the metagabbro. (a) Ca–Na-pyroxene grows first statically replacing magmatic pyroxene relics; Ca–Na-pyroxene later recrystallizes as subgrains (uppermost part of the picture). Na-amphibole overgrows Ca–Na-pyroxene. (b) Porphyroclast of magmatic pyroxene replaced by Ca–Na-pyroxene and by later Na-amphibole. (c) Fold hinge in a Ca–Na-pyroxene-rich layer; here pyroxene forms syn-kinematic subgrains. (d) Folds deforming the Ca–Na-pyroxene-rich and the clinozoisite-rich layers. (e) Epidote replacing Ca–Na-pyroxene. (f) Pressure shadows of glaucophane growing from the Ca–Na-pyroxene; the red box depicts the area shown in Figure 6b, where lawsonite occurs.

Figure 6. (a) Back-scattered electron (BSE) image of a garnet crystal replaced by epidote, chlorite and albite. (b) BSE image of a lawsonite crystal surrounded by albite; the dot inside the lawsonite crystal is the analysis point.
The Ca–Na-pyroxene layers are overgrown by light-green syn-kinematic fine-grained clinozoisite + minor Na-amphibole (2–3) and white mica (phengite) (Fig. 5d, e). The clinozoisite-rich layers preserve rare garnet that is overgrown by Fe-epidote, glaucophane and chlorite (Fig. 6a).
The blue layers consist of syn-kinematic Na-amphibole (2–3) + minor epidote, white mica (phengite), titanite and Fe-oxide. These layers include porphyroclasts, interpreted as former pyroxene (augite), replaced by fine-grained Na-amphibole (2–3) + clinozoisite + Fe-oxide or showing fringes of Na-amphibole (2) + white mica and Fe-oxide. Locally relict pyrite, replaced by chalcopyrite, occurs.
Rare layers of fine-grained syn-kinematic titanite, including Fe-oxide and rutile, are preserved.
One main metamorphic peak event, resulting in two assemblages controlled by rock compositional layering, is therefore recorded by the rock (1, 2; Fig. 7): (i) the first assemblage is defined by the static crystallization of Ca–Na-pyroxene (1) + garnet (1) + rutile (1) ± white mica (1) ± Na-amphibole (1) ± lawsonite; (ii) the second one consists of syn-kinematic Ca–Na-pyroxene (2) + garnet (2) + Na-amphibole (2) + rutile (2) + white mica (2) + lawsonite.

Figure 7. Phase crystallization diagram showing the minerals stability along the metamorphic path. Eclo – eclogitic facies; Bs – blueschist facies; the progressive numbers represent the parageneses; D1 and D2 are the two main deformational events.
These assemblages are replaced by retrograde syn-kinematic Na-amphibole (3) + epidote + white mica (3) + lawsonite + titanite.
The later crystallization event is marked by chlorite, albite and Ca-amphibole growing at the expense of garnet, Ca–Na-pyroxene and Na-amphibole.
5. Mineral chemistry
We analysed the chemical compositions of the main minerals from the lawsonite-bearing eclogitic metagabbro with an Electron Microprobe (JEOL 8200 Super Probe) at ‘Ardito Desio’ Earth Sciences Department (University of Milan, Italy), using a WDS system. The working conditions were set at 15 kV accelerating voltage and 4.9 nA beam current. Natural minerals were used as standards. Table 1 groups the analyses of representative minerals.
Table 1. Chemical analyses of representative minerals

5.a. Amphibole and pyroxene
Amphiboles are present in different proportions both in the green and blue layers. Amphibole chemical analyses have been recalculated on the basis of 24 oxygens and classified as glaucophane and actinolite, according to Hawthorne et al. (Reference Hawthorne, Oberti, Harlow, Maresch, Martin, Schumacher and Welch2012) (Table 1). Glaucophane is found as inclusions in garnet and stable along the main foliation, finally replacing omphacite; Si ranges between 7.98 and 8 atoms per formula unit (a.p.f.u.) and Altot varies in the range 1.61–1.90 a.p.f.u. The Ca-amphibole actinolite overgrows garnet, omphacite and glaucophane; Si ranges between 7.55 and 8 a.p.f.u. and Altot varies in the range 0.09–0.29 a.p.f.u.
Pyroxenes have been classified following Morimoto et al. (Reference Morimoto, Fabries, Ferguson, Ginzburg, Ross, Seifert, Zussman, Aoki and Gottardi1988) (Fig. 8a, b; Table 1); relict magmatic Ca-pyroxenes are augites and are in the order of about 1.5–2 mm in size; Na/(Na+Ca) = 0.04–0.05. Ca–Na varieties are omphacite and aegirine–augite that grow either on augite, or as fine-grained crystals (≈150–200 μm sized) along the foliation; in these pyroxenes Na/(Na+Ca) = 0.26–0.54.
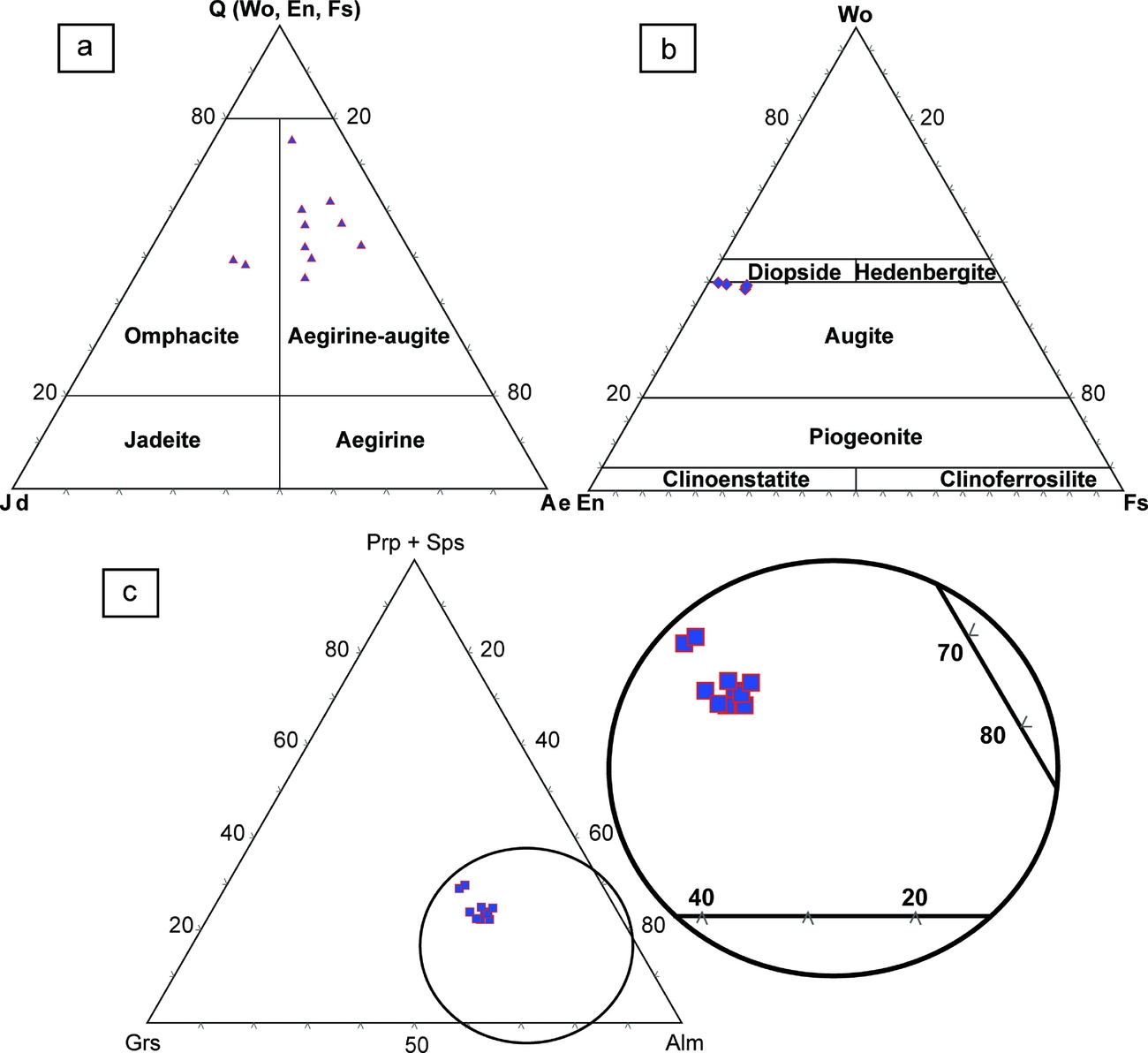
Figure 8. Classification diagrams of representative minerals. (a, b) Na-+Ca–Na- and Ca–Mg–Fe-pyroxene, respectively (after Morimoto et al. Reference Morimoto, Fabries, Ferguson, Ginzburg, Ross, Seifert, Zussman, Aoki and Gottardi1988). (c) Garnet classification diagram.
5.b. Garnet and epidote
Garnet (≈200–250 μm sized) is almandine-rich (Fig. 8c) and shows glaucophane inclusions (≈10–15 μm sized) (Fig. 6a). It is compositionally zoned with decreasing Fe and increasing Mn from the core (XFe2+= 53; XMn= 18) to the rim (XFe2+= 44; XMn= 26) (Table 1).
Epidote (≈100–150 μm sized) grows together with glaucophane (3); it has been classified on the basis of the molar fractions of the end-members in the epidote solid solutions series (clinozoisite and epidote); they were calculated respectively as: Xcz = Al(VI) − 2/((Al(VI) − 2)+Fe3+); Xep = Fe3+/((Al(VI) − 2)+Fe3+).
The chemical composition of epidote varies between the clinozoisite (Xep = 3) and the Fe-rich epidote end-members (Xep = 59) (Table 1).
5.c. Lawsonite and white mica
Preserved lawsonite (≈10–15 μm sized) has been classified on the basis of 8 oxygens (Table 1) and shows an almost pure composition with Fe content in the range of 0.02–0.06 a.p.f.u.; it grows in pressure shadows (around Ca–Na-pyroxene) made of glaucophane and is replaced by later albite.
White mica composition has been calculated on the basis of 11 oxygens and considering iron as Fe2+. White mica occurs as fine-grained crystals of phengite with Si content ranging between 3.55 and 3.77 a.p.f.u. and (Mg+Fetot) = 0.59–0.77 a.p.f.u.; the phengite compositions lie very close to the celadonite–muscovite compositional joint, reflecting the important role of Tschermak's substitution and confirming that almost no Fe3+ occurs (Vidal & Parra, Reference Vidal and Parra2000; Groppo et al. Reference Groppo, Rolfo, Sachan and Rai2016).
6. P–T estimates
We used a thermodynamic modelling approach to assess the P–T evolution of the metagabbro body and to constrain its metamorphic peak conditions. P–T pseudosections were calculated using Perple_X (http://www.perplex.ethz.ch; Connolly, Reference Connolly1990) for the system KMnTiNCFMASH with H2O considered in excess (aH2O = 1) (Table 2).
Table 2. Bulk-rock compositions of sample SC37 (lawsonite-bearing metagabbro)

The bulk-rock composition of the rock was determined by inductively coupled plasma mass spectrometry (ICP-MS) analysis at Activation Laboratories Ltd (Ontario, Canada). The thermodynamic dataset and equation of state for H2O–CO2 fluid of Holland & Powell (Reference Holland and Powell1998, revised 2002) were implemented. We used the following solid solutions: garnet (Holland & Powell, Reference Holland and Powell1998), amphibole (Wei & Powell, Reference Wei and Powell2003; White, Powell & Phillips, Reference White, Powell and Phillips2003), omphacite (Holland & Powell, Reference Holland and Powell1996), chlorite (Holland, Baker & Powell, Reference Holland, Baker and Powell1998), phengite (Holland & Powell, Reference Holland and Powell1998) and epidote (Holland & Powell, Reference Holland and Powell1998).
Since the used analytical method for defining the bulk-rock composition cannot estimate the real proportion between ferrous and ferric iron, and in order to account for the occurrence of epidote, we simulated pseudosections with various hypothetical Fe3+ amounts (i.e. Fe2O3 = 10% – 20% Fetot). The compositional isopleths calculated for garnet cores in the pseudosections with Fe2O3 = 10% – 20% Fetot diverged from each other suggesting that the considered bulk composition did not fit the rock composition during garnet growth. We thus neglected Fe3+ and simulated the pseudosection shown in Figure 9a and Figure S1 (online Supplementary Material available at http://journals.cambridge.org/geo) that includes tri- to esavariant fields. The compositional isopleths calculated for garnet cores (XFe2+ = 0.53, XCa = 0.25, XMn = 0.18, XMg = 0.04) in the free-Fe3+ pseudosection define their growth conditions in the range 465–477°C and 20.9–24.4 kbar and cross-cut into the field where chl+Ca–Na-px+wm+2 Na-amph+grt+lws+rt occur (the mineral abbreviations are after Kretz, Reference Kretz1983, except for Ca–Na-px = Ca–Na-pyroxene, Na-amph = Na-amphibole and wm = white mica). This confirms that the amount of Fe3+ in the rock is closer to 0% Fetot than to 10% Fetot. The compositional isopleths of Si in mica (Si = 3.70–3.75 a.p.f.u.) fit the isopleths of the garnet core testifying to the growth of high-pressure mica. In the pseudosection, the amount of chlorite at the P–T conditions defined by garnet growth is 13 wt%. The occurrence in our sample of chlorite stable at peak metamorphic conditions is difficult to estimate, since chlorite recrystallized at lower P–T conditions, probably erasing its former stability relations. The mineralogical association described in the above field, however, well represents the metamorphic peak assemblage that we observe in the rock; this field also includes lawsonite, which is presently observed in the Na-amphibole pressure shadows growing from Ca–Na-pyroxene. Since the garnet chemical zonation is minor, we think that the fractionation effect on the bulk composition of the system is very low; we thus used the composition of the garnet rim to trace the P–T evolution of the rock. The compositional isopleths of the garnet rim (XFe2+ = 0.44, XCa = 0.27, XMn = 0.26, XMg = 0.03) constrain the growing conditions at about 448–465°C and 19.3–22.8 kbar, at lower P–T compared to the one predicted by the garnet core.

Figure 9. P–T pseudosections in the KMnTiNCFMASH system. The mineral abbreviations are after Kretz (Reference Kretz1983) except for: cpx – clinopyroxene; Na-amph – Na-amphibole; Ca-amph – Ca-amphibole; wm – white mica; and Ca–Na-px – Ca–Na-pyroxene. Narrow and small fields have been ignored for the sake of clarity. (a) P–T pseudosection with H2O in excess. The stability conditions of the garnet core and rim are shown by green and red fields, respectively. The dotted light blue lines are isopleths of Si in white mica. The arrow shows the first stage of the retrograde path along a 3°C km−1 gradient. (b) P–T pseudosection with under-saturated conditions (H2O = LOI). The peak metamorphic conditions are highlighted by the green circle. The blue arrow depicts the later stages of the retrograde path with coexisting lawsonite and epidote.
The P–T fields derived from the stability conditions of the garnet core and rim (Fig. 9a) define a possible initial retrogression path of the eclogitic metagabbro body (arrow in Fig. 9a) along a very low thermal gradient (3°C km−1). This value (i.e. 3°Ckm−1) results from the thermobaric gradient of the path later transformed, according to the lithostatic pressure (0.3 kbar km−1), into a thermal gradient.
The later retrogressive stages are defined in our rock by the coexistence of lawsonite, Na-amphibole and epidote; this assemblage is, however, not represented in the fields of the pseudosection that we calculated with H2O in excess. To understand the possible role of H2O in the stability of this retrograde assemblage, we calculated the stability fields for the free-Fe3+ bulk-rock composition along the geotherm defined for the above exhumation path (i.e. 3°C km−1). The P/T X(H2O) pseudosection (Fig. S2, online Supplementary Material available at http://journals.cambridge.org/geo) shows that, in order to obtain the simultaneous stability of lawsonite and epidote, under-saturated conditions should occur. The transition from H2O in excess (at peak metamorphic conditions) to under-saturated conditions (during the retrograde path) could be explained with H2O request by lawsonite during its growth; the subtraction of H2O from the system (i.e. under-saturated system) allowed the simultaneous occurrence of lawsonite and epidote in the system as observed by Poli & Schmidt (Reference Poli and Schmidt1997). This has been extensively evaluated using pseudosections by Ballevre, Pitra & Bohn (Reference Ballevre, Pitra and Bohn2003), López-Carmona, Pitra & Abati (Reference López-Carmona, Pitra and Abati2013), López-Carmona et al. (Reference López-Carmona, Abati, Pitra and Lee2014) and Groppo et al. (Reference Groppo, Rolfo, Sachan and Rai2016).
The P-X(CO2) pseudosection (Fig. S3, online Supplementary Material available at http://journals.cambridge.org/geo), calculated at T = 400°C, reveals that lawsonite is stable in the range P = 11–25 kbar, for X(CO2) < 0.015 (with X(CO2) = CO2/(CO2+H2O)); for X(CO2) in the range 0.0013–0.015 lawsonite occurs together with carbonates.
Since in our samples we observed the sole presence of lawsonite (without carbonates), according to the above P-X(CO2) pseudosection, X(CO2) in the fluid should be < 0.13% of the total loss on ignition (LOI). To trace the retrograde exhumation path we thus neglected CO2 and simulated an under-saturated system considering the LOI (LOI = 4.33 wt%) as pure water (Fig. 9b and Fig. S4 in the online Supplementary Material available at http://journals.cambridge.org/geo).
The path in Figure 9b passes through fields where lawsonite coexists with epidote, crossing the rutile breakdown reaction (P < 15 kbar).
The metagabbro body thus experienced different H2O contents: at eclogitic metamorphic peak conditions (T = 465–477°C and P = 20.9–24.4 kbar), H2O was continuously supplied to the system, allowing lawsonite formation; the retrograde history was marked by the decrease of water content and under-saturated conditions, with the occurrence of both lawsonite and epidote.
7. Discussion
7.a. Occurrence of a lawsonite-bearing eclogitic metagabbro in the Voltri Massif
The lawsonite-bearing eclogitic metagabbro (Ca–Na-px+garnet+gln+rt+ph+lws) studied in this paper is an unusual lithology, as lawsonite is rarely preserved in a strongly deformed rock that records high-pressure metamorphic peak conditions, as the studied metagabbro is. Davis & Whitney (Reference Davis and Whitney2006) and Vitale Brovarone et al. (Reference Vitale Brovarone, Groppo, Hetényi, Compagnoni and Malavieille2011) in fact proposed that fresh lawsonite eclogites are preserved only in domains unaffected by post-eclogitic deformation, which prevents high-pressure mineral retrogression; we, however, observed that lawsonite grows also along the mylonitic foliation in Ca–Na-pyroxene pressure shadows. Lawsonite-bearing eclogites are reported only in a few outcrops of the Voltri Massif: lawsonite occurs as pseudomorphs replaced by aggregates of epidote + paragonite + chlorite ± phengite in eclogitic metagabbro of the eastern sector of the Voltri Massif (i.e. Mt Pesucco outcrop, Malatesta et al. Reference Malatesta, Crispini, Federico, Capponi and Scambelluri2012a ; ‘Prato del Gatto’ and ‘Case Buzzano’ eclogitic metagabbro, Capponi & Crispini, Reference Capponi and Crispini2008 a).
In the Cascine Parasi outcrop, about 3.5 km distance from our study zone, eclogite metagabbro boulders in a tectonic mélange show high-pressure assemblages with preserved stable lawsonite (Federico et al. Reference Federico, Crispini, Scambelluri and Capponi2007 b). In the same outcrop, eclogite metagabbro and metasediment bodies include aggregates interpreted as pseudomorphs after lawsonite (Cortesogno et al. Reference Cortesogno, Forcella, Lucchetti and Rossi1981; Federico et al. Reference Federico, Crispini, Scambelluri and Capponi2007 b).
Most of the worldwide lawsonite eclogites are blocks intimately associated with serpentinites, as in our case; some of them are instead coherent units associated with blueschist, orthogneiss or paragneiss (Tsujimori et al. Reference Tsujimori, Sisson, Liou, Harlow and Sorensen2006a and references therein; Vitale Brovarone et al. Reference Vitale Brovarone, Groppo, Hetényi, Compagnoni and Malavieille2011). The assemblage observed in our lawsonite-bearing eclogitic metagabbro and the estimated metamorphic peak conditions (T = 465–477°C for P = 20.9–24.4 kbar) are comparable with what is observed in worldwide lawsonite eclogites in serpentinites; they usually preserve a general prograde assemblage with grt+omp+ lws+wm+qtz±rt±ttn±gln±chl±tlc, recording peak pressure in the range 14–30 kbar and temperature between 360 and 570°C (Tsujimori et al. Reference Tsujimori, Sisson, Liou, Harlow and Sorensen2006a ,b; Tsujimori & Ernst, Reference Tsujimori and Ernst2014 and references therein; Davis & Whitney, Reference Davis and Whitney2006). We have little evidence about the prograde path of our samples, except for glaucophane inclusions in garnet, that testify to its possible occurrence also at metamorphic peak conditions. Eclogites and eclogitic metabasalts of Alpine Corsica show metamorphic peak assemblages (grt+omp+ph+gln+lws+qtz+ttn+opq, Ravna et al. Reference Ravna, Andersen, Jolivet and De Capitani2010 and references therein; omp+lws+grt+ph+ttn±gln±act±chl, Vitale Brovarone et al. Reference Vitale Brovarone, Groppo, Hetényi, Compagnoni and Malavieille2011; abbreviations are after Whitney & Evans, Reference Whitney and Evans2010) stable at c. 390°C at 2.05 GPa for eclogites, and 340–415°C, 1.9–2.6 GPa (Ravna et al. Reference Ravna, Andersen, Jolivet and De Capitani2010) or 520±20°C and 2.3±0.1 GPa, for the eclogitic metabasalts (Vitale Brovarone et al. Reference Vitale Brovarone, Groppo, Hetényi, Compagnoni and Malavieille2011).
The peak assemblages proposed for the Roboaro lawsonite-bearing eclogitic metagabbro are particularly similar to lawsonite eclogites from South Motagua (Guatemala; Tsujimori et al. Reference Tsujimori, Sisson, Liou, Harlow, Sorensen, Hacker, McClelland and Liou2006b ), the Central Pontides (Turkey; Altherr et al. Reference Altherr, Topuz, Marschall, Zack and Ludwing2004) and the Sivrihisar Massif (Turkey; Davis & Whitney, Reference Davis and Whitney2006), even if the stability conditions are mostly comparable to the South Motagua lawsonite eclogites (1.8–2.5 GPa and 290–470°C).
The development and preservation of lawsonite in rocks that experienced high-pressure conditions requires both cold subduction and a rapid exhumation (Tsujimori et al. Reference Tsujimori, Sisson, Liou, Harlow and Sorensen2006a ,b; Çetinkaplan et al. Reference Çetinkaplan, Candan, Oberhänsli and Bousquet2008). Lawsonite is an important carrier of water and trace elements to high depths (at least 300 km in cold subduction zones): it accommodates up to 11.5 wt% of H2O that can be released from the slab, providing volatiles for arc magmatism and subduction zone seismicity (Schmidt & Poli, Reference Schmidt and Poli1998; Tsujimori et al. Reference Tsujimori, Sisson, Liou, Harlow and Sorensen2006a ,b and references therein).
The preservation of lawsonite in the rocks along the Roboaro River suggests that at least one of these conditions (i.e. cold subduction and rapid exhumation) has been verified. Concerning the first hypothesis (i.e. cold subduction), thermodynamic modelling suggests that the metagabbro body reached peak metamorphic conditions at a relatively low temperature (T = 465–477°C for P = 20.9–24.4 kbar), in a setting where H2O was continuously provided to a cold system. Spandler & Pirard (Reference Spandler and Pirard2013) suggested that the maximum P–T conditions reached by lawsonite eclogites may not necessarily reflect the general conditions of cold subduction: as the shallow fore-arc mantle is cooler than the deep sub-arc mantle wedge, the top of the subducting slab undergoes rapid heating after coupling with the hot asthenospheric mantle wedge. The preservation of lawsonite eclogite can suggest that they were exhumed prior to heating of the slab by the overlying asthenosphere and were not related to a general cold subduction.
Concerning the exhumation rate, even if a rapid exhumation has been proposed for the preservation of lawsonite in high-pressure rocks Tsujimori et al. Reference Tsujimori, Sisson, Liou, Harlow and Sorensen(2006a), Agard et al. (Reference Agard, Amato, Jolivet and Burov2009) suggested that lawsonite can survive at slow exhumation rates if refrigerant conditions are maintained. Proposed exhumation rates for eclogite and blueschist rocks of the Voltri Massif range from 3.3–3.9 mm y−1 (Federico et al. Reference Federico, Capponi, Crispini, Scambelluri and Villa2005) to 25 mm y−1 (Rubatto & Scambelluri, Reference Rubatto and Scambelluri2003); the latter velocity has been recalculated by C. Malatesta (unpub. Ph.D. thesis, Univ. degli Studi di Genova, 2011) and attests to values of 10–12 mm y−1, considering an exhumation time of 4 Ma. The exhumation rates proposed for the Voltri Massif are therefore in general low values and seem to confirm that lawsonite is preserved thanks to cold conditions during subduction rather than a rapid exhumation.
7.b. CO2-rich fluids and fluid–rock interaction
The occurrence of carbonated serpentinites, wrapping the eclogitic metagabbro and metasediment bodies, suggests that an intense and long-lasting circulation of CO2-rich fluids affected the area around the Roboaro River and the adjacent zones. The country-rock serpentinites show various degrees of carbonation until their complete transformation into listvenites. Serpentinites are replaced by carbonate minerals starting preferentially from veins that either follow the pervasive foliation or from mesh structures; carbonates also grow along late fracture systems. All these structures acted as preferential pathways for the infiltration of CO2-bearing fluids during subduction.
The occurrence of listvenites and carbonated serpentinites mainly along fault zones (Fig. 1b) also confirms the structural control of fluid circulation along shear zones and the damage zone of faults.
Further evidence on the circulation of CO2-rich fluids comes from an outcrop some hundreds of metres from the Roboaro River study area (i.e. La Pesca locality). At this site, Cortesogno, Galbiati & Principi (Reference Cortesogno, Galbiati and Principi1980), Cortesogno, Lucchetti & Massa (Reference Cortesogno, Lucchetti and Massa1981) and Scambelluri et al. (Reference Scambelluri, Bebout, Belmonte, Gilio, Campomenosi, Collins and Crispini2016) described a garnet-bearing marble interlayered with serpentinite, dolomite and hybrid-rocks (the latter intermediate between serpentinite and marble). These rocks have been interpreted either as ophicarbonate bodies formed on an ancient ocean-floor (Cortesogno, Galbiati & Principi, Reference Cortesogno, Galbiati and Principi1981) or as a serpentinite–marble contact; in both cases these rocks were highly deformed and metamorphosed during the high-pressure subduction history (Scambelluri et al. Reference Scambelluri, Bebout, Belmonte, Gilio, Campomenosi, Collins and Crispini2016). Studying these rocks, Scambelluri et al. (Reference Scambelluri, Bebout, Belmonte, Gilio, Campomenosi, Collins and Crispini2016) hypothesized that CO2 fluid circulation, and therefore the formation of high-P ophicarbonate, was linked to the dehydration of serpentinite close to metamorphic peak conditions; the released aqueous fluids triggered the breakdown of dolomite in the nearby marbles and this reaction discharged C into the fluids.
In the Roboaro River study area, we have no direct analogies with the outcrop cited above and therefore we do not have clear constraints about the timing of CO2 fluid circulation into the serpentinites.
No evidence (e.g. carbonates, carbonate-bearing veins) of a strong interaction of the CO2-rich fluids with the lawsonite-bearing eclogitic metagabbro at high-pressure conditions occurs; this is in accordance with the results of the pseudosections calculation (Section 6; Fig. S4, online Supplementary Material available at http://journals.cambridge.org/geo), suggesting that lawsonite is stable in the system for very low contents of CO2 (i.e. X(CO2) < 0.13 wt%).
At the current state of knowledge of the area, the reasons why the lawsonite-bearing eclogitic metagabbro escaped from an intense CO2 fluid circulation are still puzzling; nonetheless some reasonable scenarios could be envisaged: (i) the lawsonite-bearing eclogitic metagabbro and host-rocks have contrasting rheologies and permeabilities; (ii) the lithotypes (i.e. eclogitic metagabbro versus serpentinite) are characterized by different chemical affinities with CO2-bearing fluids; (iii) the CO2-bearing fluid circulation pre-dated the coupling of the lawsonite-bearing eclogitic metagabbro with serpentinite.
7.c. Geodynamic implications
The area along the Roboaro River is an example of a hydrated slab portion where both aqueous fluids and carbonate-rich fluids were active. This area of the Voltri Massif recorded multiple superposed deformations, related to a general shear regime. The alternation of Na-amphibole-rich and Ca–Na-pyroxene-rich layers in the eclogitic metagabbro body and the concurrent interfolding of the metagabbro with the metasediment lens and serpentinite are one of the most evident effects of deformation (Fig. 10).

Figure 10. Schematic model of the progressive deformation of the study area. (a) Stage 1: metagabbro and metasediment lenses surrounded by serpentinites with circulating fluids. (b) Stage 2: Progressive deformational events linked with the metamorphic peak and exhumation stages that produced the alternating blue and green layering. (c) Stage 3: Later stretching of the metagabbro body that caused the boudinage of the green layer. (d) Interpretative schematic geological cross-section through the lawsonite-bearing metagabbro body.
Na-amphibole-rich and Ca–Na-pyroxene-rich layers could derive, respectively, from the hydrous rim and the anhydrous core of mafic lenses incorporated inside serpentinite country-rock, as suggested for metabasalts of the Zermatt-Saas ophiolite by Angiboust & Agard (Reference Angiboust and Agard2010). At the present stage, it is not possible to assess if the amphibole-rich rim reflects an oceanic stage of hydration of the outermost rim of mafic bodies inside the ultramafic serpentinized basement, or a later interaction of the boudinaged mafic bodies with fluids during the subduction path.
The ductile shear regime that affected this area strongly acted at least from eclogite-facies metamorphic conditions inside the subduction zone, deforming the Ca–Na-pyroxene + garnet association in the metagabbro, and continued to be active at blueschist-facies conditions, during the exhumation stage. The shear regime also enhanced the circulation of CO2-rich fluids through the country-rock serpentinite, triggering its partial to total carbonation and transformation into listvenite. The following brittle–ductile structures affecting the metagabbro (i.e. boudinage and low-angle shearing surfaces) formed during late-stage greenschist-facies conditions.
The occurrence of preserved lawsonite coupled with the petrological study suggest that this area underwent different P–T conditions (lower T) compared to most of the Voltri Massif, where eclogite metagabbros record in general a peak temperature higher than 500°C (Scambelluri et al. Reference Scambelluri, Hoogerduijn Strating, Piccardo, Vissers and Rampone1991; Federico et al. Reference Federico, Capponi, Crispini and Scambelluri2004; Vignaroli et al. Reference Vignaroli, Rossetti, Bouybaouene, Massonne, Theye, Faccenna and Funiciello2005); exceptions are the eclogites studied by Liou et al. (Reference Liou, Zhang, Ernst, Liu and McLimans1998) and Brouwer, Vissers & Lamb (Reference Brouwer, Vissers and Lamb2002), that record T = 450°C for P = 18 kbar, and the eclogite studied by Malatesta et al. (Reference Malatesta, Crispini, Federico, Capponi and Scambelluri2012a ), with peak T = 460–500°C and P = 22–28 kbar; in all these cases, however, lawsonite is absent or is destabilized and occurs as pseudomorphs, suggesting that these eclogites followed hotter exhumation paths.
Accordingly to what was proposed by Spandler & Pirard (Reference Spandler and Pirard2013) for lawsonite-bearing high-pressure rocks, this area could represent a portion of the top of the subducted slab, close to the plate interface: this slab portion was coupled with a hydrated and ‘cool’ mantle wedge that kept our eclogitic metagabbro in a low-temperature regime with the preservation of lawsonite.
The different P–T paths identified for the eclogites of the Voltri Massif confirm that it is made up by rock bodies subducted to various depths and brought to the surface along different exhumation trajectories. Moreover, the occurrence of rocks with different paleogeographic origins (e.g. ocean versus continent) in a serpentinite matrix requires a large-scale mechanism that could associate them. As suggested by Federico et al. (Reference Federico, Crispini, Scambelluri and Capponi2007 a) and Malatesta et al. (Reference Malatesta, Crispini, Federico, Capponi and Scambelluri2012a ,b), the geodynamic mechanism responsible for these kinds of assemblages and their evolution could be involvement in a low-viscosity serpentinite channel close to the plate interface.
8. Main remarks
This work presents a detailed structural, petrographical and petrological study of the Roboaro River area (Voltri Massif, Ligurian Western Alps). The occurrence of a lawsonite-bearing eclogite folded together with metasediments and variously carbonated serpentinites allowed us to interpret this area as an example of a highly hydrated slab portion, where both aqueous fluids and carbonate-rich fluids were present at high-pressure conditions.
The lawsonite-bearing metagabbro body reached eclogitic metamorphic peak conditions at T = 465–477°C and P = 20.9–24.4 kbar, with H2O continuously supplied to the system. The exhumation path was characterized by H2O under-saturated conditions, with the occurrence of both lawsonite and epidote.
The low temperature recorded by the lawsonite-bearing eclogitic metagabbro body led us to interpret this area as a portion of the top of the subducted slab coupled with the hydrated and ‘cool’ mantle wedge.
Both the multiple deformations recorded during the whole studied history of the body and the occurrence in the area of rocks with different paleogeographic origins (e.g. ocean versus continent), wrapped in a serpentinite matrix, witness that this sector was close to the slab–mantle interface that evolved in a shear regime. These rocks were merged within a low-viscosity serpentinite channel that brought them back to the surface.
Acknowledgements
We greatly appreciate the discussion with Laura Crispini, Laura Federico and Giovanni Capponi; we acknowledge Nicholas Vaiarini for the field assistance. We also thank Andrea Festa, Alicia López-Carmona and Idael Francisco Blanco-Quintero for their detailed and constructive comments which improved this manuscript. This work was financially supported by PRIN 2010 project ‘Nascita e morte dei bacini oceanici: processi geodinamici dal rifting alla collisione continentale negli Orogeni mediterranei e circum-mediterranei’, national Coordinator G. Capponi.
Supplementary material
To view supplementary material for this article, please visit https://doi.org/10.1017/S0016756817000395.