1. Introduction
Ophiolites represent oceanic remnants in mountain chains and their tracking, together with the reconstruction of their structural and metamorphic history, is still one of the basic tools for understanding the dynamics of collisional chain development. In the Alps, the key significance of ophiolites in geodynamics was long understood before the birth of plate tectonics (e.g. Brongniart, Reference Brongniart1813; Argand, Reference Argand1911; Steinmann, Reference Steinmann, Dilek and Newcomb1927). Since the first geological research within the Alps ophiolites from the Piedmont zone have been a favourable investigation object (Dal Piaz, Reference Dal Piaz2010), especially in the exploration of deformation and mineral transformation histories of high-pressure (HP) and ultra-high-pressure (UHP) units of the Zermatt-Saas Zone (ZSZ). In the last years a debate developed on this portion of the Piedmont zone, regarding whether the pressure–temperature (P–T) conditions registered by ZSZ rocks are largely uniform or if different portions of ZSZ recorded different P–T-peak conditions and underwent different exhumation paths over different time spans (e.g. Lapen et al. Reference Lapen, Johnson, Baumgartner, Mahlen, Beard and Amato2003; Li, Rahn & Bucher, Reference Li, Rahn and Bucher2004; Angiboust et al. Reference Angiboust, Agard, Jolivet and Beyssac2009; Groppo, Beltrando & Compagnoni, Reference Groppo, Beltrando and Compagnoni2009; Angiboust & Agard, Reference Angiboust and Agard2010; de Meyer et al. Reference de Meyer, Baumgartner, Beard and Johnson2014; Skora et al. Reference Skora, Mahlen, Johnson, Baumgartner, Lapen, Beard and Szilvagyi2015; Weber & Bucher, Reference Weber and Bucher2015; Weber et al. Reference Weber, Sandmann, Miladinova, Fonseca, Froitzheim, Münker and Bucher2015). This last view has been recently reinforced by multiscalar structural and petrological analysis supported by detailed field mapping (Rebay, Spalla & Zanoni, Reference Rebay, Spalla and Zanoni2012; Weber & Bucher, Reference Weber and Bucher2015; Zanoni, Rebay & Spalla, Reference Zanoni, Rebay and Spalla2016). The multiscalar analytical approach could be deeply reinforced by careful geochronological work, and becomes important in understanding the critical size of oceanic units able to record a homogeneous structural and metamorphic evolution during burial and exhumation paths in a subduction system. This means identifying volumes that have completed, remaining intact, their tectonic trajectory during a given time span. In addition, this allows light to be shed on how the coupling and decoupling of tectonic units can occur in the subduction mantle wedge or in the orogenic wedge during continental collision (e.g. Cloos, Reference Cloos1982; Chemenda et al. Reference Chemenda, Mattauer, Malavieille and Bokun1995; Stoeckhert & Gerya, Reference Stoeckhert and Gerya2005; Baumann, Gerya & Connolly, Reference Baumann, Gerya, Connolly, Spalla, Marotta and Gosso2010; Roda, Marotta & Spalla, Reference Roda, Marotta and Spalla2010; Gerya, Reference Gerya2011; Malatesta et al. Reference Malatesta, Crispini, Federico, Capponi and Scambelluri2012, Reference Malatesta, Gerya, Crispini, Federico and Capponi2016; Roda, Spalla & Marotta, Reference Roda, Spalla and Marotta2012). Different subduction dynamics can result in the formation of tectonic units with different sizes and different ages, sharing a coherent structural and metamorphic evolution.
The main goal of this contribution is to present new age data on microstructural sites selected on the basis of a well-defined chronology of superimposed structures and metamorphic imprints at the regional scale, and to implement the chronological outline of eclogitized ZSZ ophiolites from the upper Valtournanche serpentinites in order to take some steps towards a better constrained regional framework.
2. Geological setting
The ZSZ, together with the Combin Zone (CZ), represents a Tethyan ocean relict that comprises rocks variously affected by oceanic- and subduction-related metamorphism, which now are transposed along the axial belt of the Western Alps (Caron et al. Reference Caron, Polino, Pognante, Lombardo, Lardeaux, Lagabrielle, Gosso and Allenbach1984; Reddy, Wheeler & Cliff, Reference Reddy, Wheeler and Cliff1999; Dal Piaz, Reference Dal Piaz2010; Spalla et al. Reference Spalla, Gosso, Marotta, Zucali and Salvi2010). These two zones are tectonically sandwiched between the continental Monte Rosa (beneath) and Dent Blanche – Sesia Lanzo nappes (above) that belong to the Penninic and Austroalpine domains, respectively (e.g. Bearth, Reference Bearth1967; Polino, Dal Piaz & Gosso, Reference Polino, Dal Piaz and Gosso1990). The ZSZ (Fig. 1a, b) underlies the CZ and, due to their different lithostratigraphic and metamorphic features, these two units have been interpreted as deriving from the oceanic lithosphere and from the ocean–continent transition zone, respectively (e.g. Dal Piaz & Ernst, Reference Dal Piaz and Ernst1978; Dal Piaz et al. Reference Dal Piaz, Venturelli, Spadea and Di Battistini1981). In the upper Valtournanche, ZSZ and CZ are separated by slices of pre-Alpine continental rocks and schists deriving from Permian–Cretaceous sediments (Dal Piaz, Reference Dal Piaz, Gosso, Jadoul, Sella and Spalla1999, Reference Dal Piaz2010). The main difference between ZSZ and CZ is the dominant metamorphic imprint: eclogite facies in the first and greenschist facies in the second. Despite the pervasive greenschist re-equilibration, CZ rocks preserve blueschist facies relicts, whereas remnants of eclogite facies assemblages have never been detected, even in the less-deformed rocks (Cartwright & Barnicoat, Reference Cartwright and Barnicoat2002; Dal Piaz, Reference Dal Piaz1974; Dal Piaz & Ernst, Reference Dal Piaz and Ernst1978; Ring, Reference Ring1995). The greenschist facies assemblages are extremely localized in the mostly eclogitized rocks of ZSZ and are mainly interpreted as exhumation-related metamorphic imprints (e.g. Cartwright & Barnicoat, Reference Cartwright and Barnicoat2002; Ernst & Dal Piaz, Reference Ernst and Dal Piaz1978); scattered relics pre-dating the eclogite facies peak preserve blueschist facies mineral assemblages (e.g. Chinner & Dixon, Reference Chinner and Dixon1973; Ernst & Dal Piaz, Reference Ernst and Dal Piaz1978). Metabasic rocks are the most explored to determine the Alpine metamorphic evolution of this portion of the Tethys ocean and results give a wide span of P–T values in different portions of ZSZ: 2.5–3.0 GPa and 550–600°C (Bucher et al. Reference Bucher, Fazis, de Capitani and Grapes2005); 1.9–2.2 GPa and 530–600°C (Dale et al. Reference Dale, Burton, Pearson, Gannoun, Alard, Argles and Parkinson2009); and, south of the Aosta-Ranzola fault (St Marcel valley), 2.1±0.3 GPa and 550±60°C (Martin et al. Reference Martin, Rebay, Kienast and Mevel2008). The metagabbros of the Swiss portion of the ZSZ record peak conditions of 2.5–2.8 GPa and 600–610°C (Bucher & Grapes, Reference Bucher and Grapes2009) or alternatively 1.9–2.2 GPa and 500–580°C (Dale et al. Reference Dale, Burton, Pearson, Gannoun, Alard, Argles and Parkinson2009). Pressures from 2.7 to >3.2 GPa and temperatures of 590–630°C are estimated at Lago di Cignana (e.g. Reinecke, Reference Reinecke1991, Reference Reinecke1998; van der Klauw, Reinecke & Stöckhert, Reference van der Klauw, Reinecke and Stöckhert1997; Groppo, Beltrando & Compagnoni, Reference Groppo, Beltrando and Compagnoni2009), where microdiamonds have also been found in oceanic metasedimentary rocks (Frezzotti et al. Reference Frezzotti, Selverstone, Sharp and Compagnoni2011). Peak P–T estimates from serpentinites of the Swiss side are at 2.0–2.5 GPa and 600–650°C (Li, Rahn & Bucher, Reference Li, Rahn and Bucher2004), whereas in the Italian side they are at 2.2–2.8 GPa and 580–620°C (Rebay, Spalla & Zanoni, Reference Rebay, Spalla and Zanoni2012). The latter estimates have recently been supported by P–T peak conditions determined by thermodynamic modelling of the hosted eclogitized rodingites (2.3–2.8 GPa and 580–660°C; Zanoni, Rebay & Spalla, Reference Zanoni, Rebay and Spalla2016). All these P–T estimates led to the inference of trajectories indicating the occurrence of heterogeneous metamorphic evolutions in different portions of ZSZ. In contrast, different authors have suggested a homogeneous evolution for the entire ZSZ based on the assemblages from metabasites and calcschists, with peak conditions at 2.3±0.1 GPa and 540±40°C (Angiboust et al. Reference Angiboust, Agard, Jolivet and Beyssac2009; Angiboust & Agard, Reference Angiboust and Agard2010). In addition, part of the Monviso ophiolite has been interpreted as forming a single unit with the ZSZ, which would consist of a continuous slab, recording a coherent metamorphic evolution during the Alpine subduction (Angiboust et al. Reference Angiboust, Langdon, Agard, Waters and Chopin2012). This interpretation differs from that of previous authors, suggesting that the Monviso is a complex of eclogitized ophiolitic slices accreted during the final stages of their exhumation on the basis of contrasted P–T trajectories (Schwartz et al. Reference Schwartz, Lardeaux, Guillot and Tricart2000; Guillot et al. Reference Guillot, Schwartz, Hattori, Auzende and Lardeaux2004). Consistent with a heterogeneous metamorphic evolution, Alpine ages indicate a wide time interval of re-equilibration during subduction of the ZSZ rocks and associated continental slivers (Table 1) of 80–38 Ma, with ages interpreted as referred to peak conditions as 52–38 Ma (Bowtell, Cliff & Barnicoat, Reference Bowtell, Cliff and Barnicoat1994; Rubatto, Gebauer & Fanning, Reference Rubatto, Gebauer and Fanning1998; Dal Piaz et al. Reference Dal Piaz, Cortiana, Del Moro, Martin, Pennacchioni and Tartarotti2001; Skora et al. Reference Skora, Lapen, Baumgartner, Johnson, Hellebrand and Mahlen2009, Reference Skora, Mahlen, Johnson, Baumgartner, Lapen, Beard and Szilvagyi2015; Springer et al. Reference Springer, Lapen, Baumgartner, Johnson and Beard2009; de Meyer et al. Reference de Meyer, Baumgartner, Beard and Johnson2014; Weber et al. Reference Weber, Sandmann, Miladinova, Fonseca, Froitzheim, Münker and Bucher2015). Moreover, considering only U–Pb zircon methods, such ages cluster at c. 48–40 Ma (Table 1). Protolith ages indicate gabbro emplacement at 167–161 Ma and sediment deposition during 170–150 Ma (Table 1; Rubatto, Gebauer & Fanning, Reference Rubatto, Gebauer and Fanning1998).
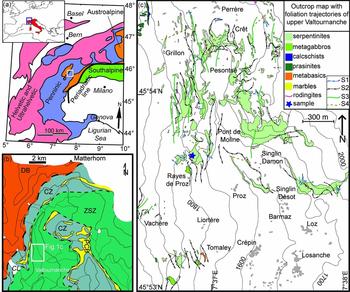
Figure 1. Geological and structural outline of the upper Valtournanche. (a) Tectonic sketch map of the Western Alps and its location within Europe and the Mediterranean region. (b) Interpretative tectonic sketch of the upper Valtournanche redrawn from the geotectonic map of the Aosta Valley (De Giusti et al. Reference De Giusti, Dal Piaz, Schiavo, Massironi, Monopoli and Bistacchi2003); area indicated by the thick white square in (a). ZSZ – Zermatt-Saas Zone; PCB – Pancherot-Cime Bianche-Bettaforca unit; CZ – Combin Zone; DB – Dent Blanche nappe; CL – Cignana Lake. (c) Outcrop map with the foliation trajectories of the metaophiolites of the upper Valtournanche (ZSZ), synthesized from an original mapping (unpublished) at 1:5000 scale. Relative chronology of superimposed foliation is shown in the legend. Topography redrawn from the technical map of the Val d'Aosta Regional Administration without hydrography.
Table 1. Summary of age data for Western Alps ophiolites and peak P–T conditions for Zermatt-Saas Zone
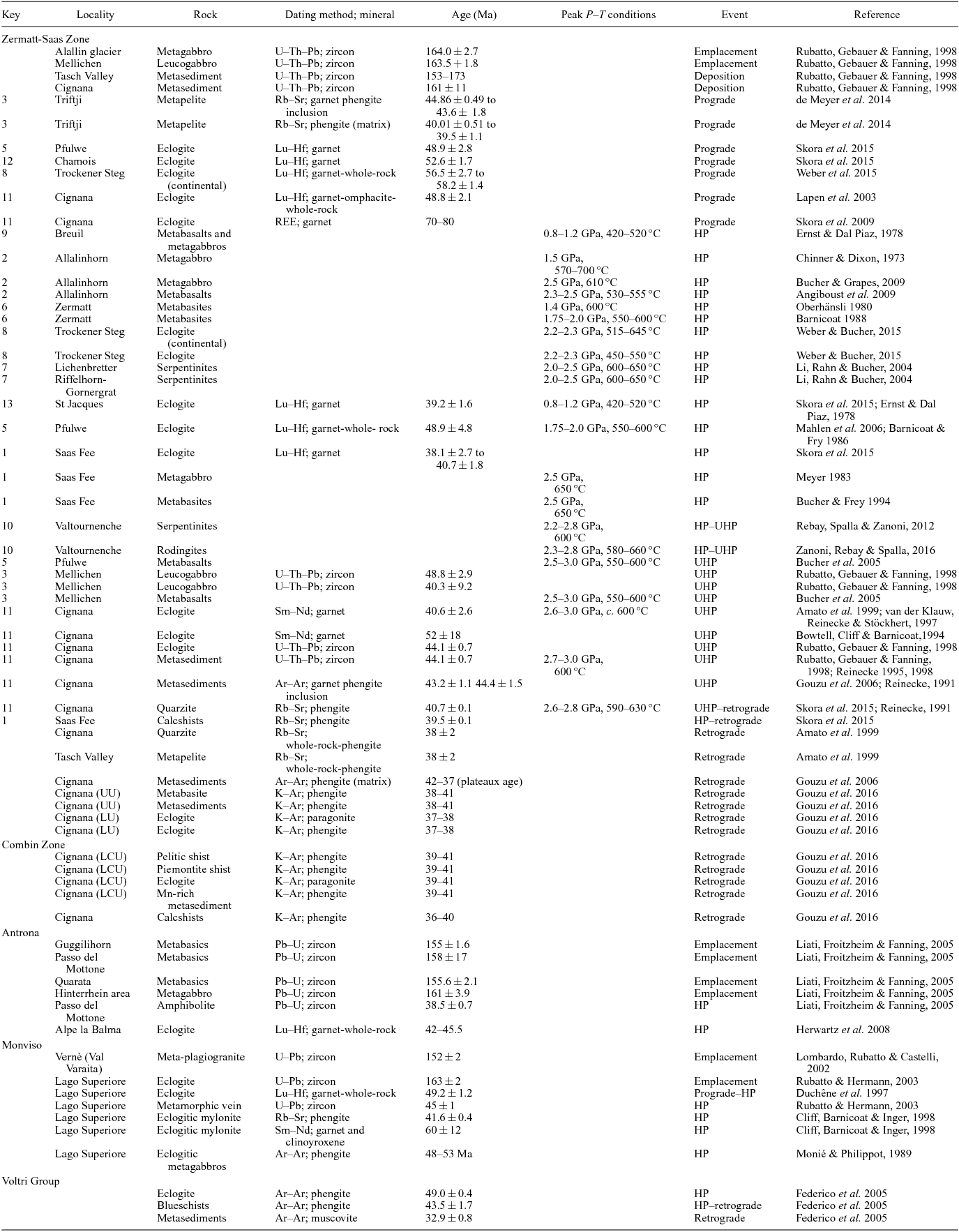
3. Mesostructure
Along the upper Valtournanche valley, between Valtournanche village and Perrère hydroelectric plant, serpentinite is the dominant lithotype. Hectometre-sized metagabbro bodies and up to a few metres thick rodingite boudins and dykes are associated with serpentinites, together with marbles and calcschists (Fig. 1c). The dominant fabric in serpentinites, metagabbros and rodingites is the tectonitic to mylonitic S2 foliation, and less-deformed domains contain textural and structural pre-D2 relicts (Fig. 2a–c). S2 is in turn overprinted by two groups of superimposed ductile structures (D3 and D4); all ductile structures were mapped with spatial continuity (Fig. 1c) by means of multiscale structural analysis (Rebay, Spalla & Zanoni, Reference Rebay, Spalla and Zanoni2012; Zanoni et al. Reference Zanoni, Rebay, Bernardoni, Spalla, Zucali, Spalla and Gosso2012; Zanoni, Rebay & Spalla, Reference Zanoni, Rebay and Spalla2016). S2 locally is mylonitic and associated with a tight to isoclinal folding that is characterized by a shallow west-dipping axial plane and a NNW–SSE shallow plunging axis. Contacts between serpentinites and metagabbros or rodingites are mainly reworked during the S2 development (see Zanoni, Rebay & Spalla, Reference Zanoni, Rebay and Spalla2016). In serpentinite, S2 is marked by Alpine HP–UHP mineral assemblages and is pre-dated by clinopyroxene porphyroclasts and amphibole-clinopyroxene-bearing vein nets (Fig. 2a), ascribable to the oceanic evolution, and by an S1 foliation (Fig. 2b) that represents the oldest detectable Alpine fabric (Rebay, Spalla & Zanoni, Reference Rebay, Spalla and Zanoni2012; Zanoni, Rebay & Spalla, Reference Zanoni, Rebay and Spalla2016). S1 foliation and up to millimetre-sized oceanic relicts are also preserved in rodingites (Zanoni, Rebay & Spalla, Reference Zanoni, Rebay and Spalla2016). S2 is post-dated by localized ductile shear zones and by two groups of folds, represented by tight folds with west-dipping axial plane and a N–S shallow plunging axis (D3, Fig. 2d) and by open to gentle upright folds with shallow axis (D4), respectively. Among these latter two folding stages, the first is associated with the development of a differentiated axial plane foliation S3 that in serpentinites is marked by a mineral assemblage indicating low-pressure metamorphic conditions, representing the retrograde metamorphic re-equilibration during uplift (Rebay, Spalla & Zanoni, Reference Rebay, Spalla and Zanoni2012).

Figure 2. (a) Net of whitish amphibole-clinopyroxene-bearing veins intersected by S2 foliation. Hammer for scale. (b) S1 foliation folded during the development of D2 and post-D2 deformation stages. D2 is accompanied by the development of the S2 foliation and D3 produced only a folding (D3-AP = D3 axial plane). Pencil for scale. (c) Pervasive S2 foliation that wraps around rodingite boudins. Hammer for scale. (d) Metre-sized D3 fold. Hammer for scale.
4. Microstructures and mineral chemistry
Serpentinites have been divided into two groups on the basis of D2 parageneses – Ti-clinohumite-bearing and eclogite facies serpentinite – described in detail by Rebay, Spalla & Zanoni (Reference Rebay, Spalla and Zanoni2012). Pre-D2 minerals are represented by porphyroclasts of olivine1, clinopyroxene1 and Ti-clinohumite1 that are wrapped by S2 foliation (Fig. 3a). New mineral growths occur at the rims of clinopyroxene, Ti-clinohumite and olivine as fringes elongated parallel to S2 (Fig. 3a, b). The parageneses associated with D2 consist of olivine2, clinopyroxene2, antigorite±Ti-clinohumite2±chlorite±zircon (Fig. 3a, b), whereas post-D2 foliation is defined by serpentine, chlorite±amphibole (Fig. 3c). A sample of eclogite facies serpentinite, collected north of Rayes de Proz (star in Fig. 1c), is characterized by pervasive S2 foliation, wrapping around clinopyroxene porphyroclasts (Fig. 3d–f) and relationships between mineral growth and S2 development are similar to those described above. Two generations of zircon (namely zircon1 and zircon2) are easily recognizable where pre-D2 cores consist of porphyroclasts rimmed by syn-D2 fringe-like overgrowths, aligned along S2 foliation (Fig. 3g). This texture is consistent with that of clinopyroxene, Ti-clinohumite and olivine from all serpentinite types (Fig. 3h). Larger zircon grains are at times boudinaged (Fig. 3i).

Figure 3. Microphotographs of (a–c) eclogitized and Ti-clinohumite-bearing serpentinite, and (d–i) of the zircon bearing eclogitized serpentinite sample. (a) Ti-clinohumite1 porphyroclasts (Ti-chu1) wrapped by S2 defined by Ti-clinohumite2 (Ti-chu2), olivine2 (Ol2), clinopyroxene2 (Cpx2) and antigorite (Atg); (b) syn-D2 mylonite with a clinopyroxene1 porphyroclast (Cpx1) and banded S2 foliation defined by clinopyroxene2 (Cpx2), olivine2 (Ol2) and antigorite (Atg); (c) S2 defined by antigorite overprinted by an incipient S3 crenulation cleavage; amphibole (Amph) porphyroblasts (note basal sections) show SPO parallel to S3; (d) large field view of the zircon-bearing serpentinite with a large, fractured, clinopyroxene (Cpx1) porphyroclast (dark) wrapped by S2, which is defined by antigorite (Atg), olivine2 and clinopyroxene2 (in the lower part there are five zircon porphyroclasts, Zrn); (e) clinopyroxene1 porphyroclast (Cpx1) with clinopyroxene2 (Cpx2) spikes parallel to S2, here defined by antigorite (Atg); (f) SEM backscattered image of a large clinopyroxene1 (Cpx1) with spikes of clinopyroxene2 (Cpx2) parallel to S2; (g) zircon (Zrn1) wrapped by antigorite defining S2, showing zircon2 (Zrn2) growths parallel to S2 (see zircon shape in CL image in Fig. 4c, h) clinopyroxene (Cpx) and zircon (Zrn) porphyroclasts wrapped by S2 overprinted by S3 crenulation cleavage; and (i) large zircon boudinaged within S2 that wraps a small clinopyroxene1 (Cpx1) porphyroclast, overprinted by S3 crenulation cleavage.
Compositions of olivine and clinopyroxene associated with pre-D2 and syn-D2 assemblages are different and distinctive, as detailed in Rebay, Spalla & Zanoni (Reference Rebay, Spalla and Zanoni2012). In summary, olivine cores and rims are very similar in composition, with a slight decrease in Mg towards the rims.
Clinopyroxene cores are Al- (up to 0.3 apfu), Ti- and Cr-rich and Ca-poor (0.8–0.92 apfu), whereas syn-D2 rims are Ca-rich (0.94–1 apfu) and Ti-, Cr- and Al-poor.
Syn-D2 serpentine has higher Mg (up to 11.45 apfu) and lower Fe (up to 0.9 apfu) and Al (up to 0.8 apfu) contents than pre-D2 serpentine. Based on the major-element compositional variations, using classical geothermobarometry and thermodynamic modelling, 2.5±0.3 GPa and 600±20°C were estimated for the formation of mineral parageneses associated with D2 deformation stage (Rebay, Spalla & Zanoni, Reference Rebay, Spalla and Zanoni2012).
Clinopyroxene and zircon cores were analysed for trace-element composition with laser ablation (LA) ICP-MS (Table 2) at the CNR–IGG UOS of Pavia. The instrument couples a Nd:YAG laser working at 266 nm with a quadrupole ICP-MS (Perkin Elmer - DRCe). All analyses were carried out using a 10 μm spot (see online supplementary material, available at http://journals.cambridge.org/geo, for details on the analytical method).
Table 2. Trace element composition (ppm) of clinopyroxene and zircon (zircon grain names refer to Fig. 5)
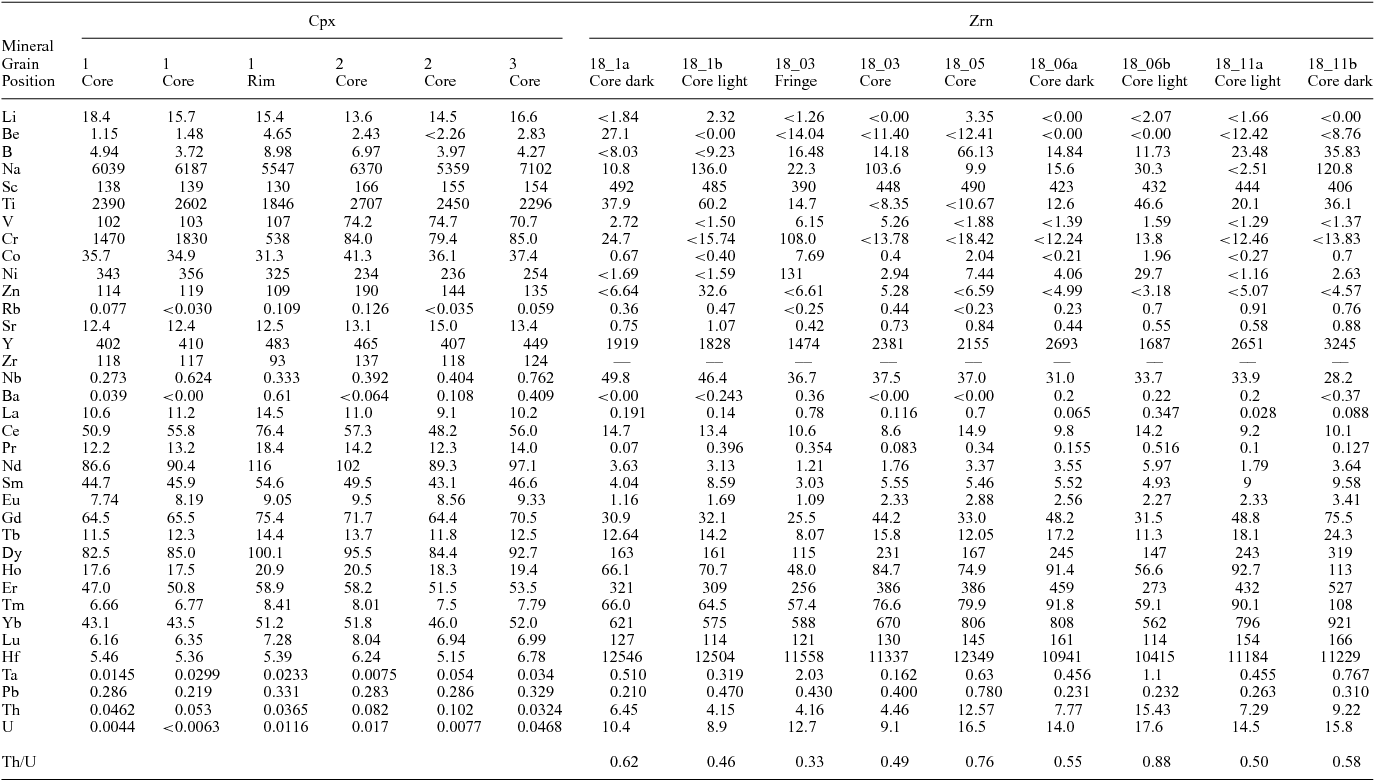
The chondrite-normalized rare Earth element (REE) pattern (Fig. 4) of clinopyroxene is characterized by flat heavy REE (at about 100 times CI chondrite), a weak light REE depletion and negative Eu anomaly. Zr and Y concentrations are relatively high and are in the ranges 93.3–118 ppm and 402–449 ppm. The trace-element composition of zircon cores is characterized by low U concentration (9–18 ppm) and a chondrite-normalized REE pattern typical of growth under magmatic conditions, characterized by light REE depletion, positive Ce anomaly and heavy REE (HREE) enrichment (up to 3000 times CI chondrite). Zircon REE patterns parallel that of clinopyroxene for the occurrence of a negative Eu anomaly. Zircon fringe dimensions allowed only a single analysis. Trace-element composition of zircon fringes matches that of magmatic cores, revealing no significant difference in both REE contents and Th/U ratios. The absence of garnet and other mineral phases with high compatibility for REE (and other trace elements) in the HP–UHP mineral paragenesis in these rocks prevents their redistribution during zircon recrystallization, and accounts for the similar concentrations between magmatic cores and metamorphic rims.

Figure 4. REE patterns (chondrite normalized) of clinopyroxene and zircon cores.
5. U–Pb zircon geochronology
5.a. Method
U–Pb zircon geochronology was carried out with LA-ICP-MS on polished thin-sections at the CNR–IGG UOS of Pavia. The system couples an ArF excimer laser microprobe (type GeoLas102 from MicroLas) with a sector field ICP-MS (type Element from ThermoFinnigan). The signal of 202Hg, 204(Pb+Hg), 206Pb, 207Pb, 208Pb, 232Th and 238U masses were acquired. 202Hg is acquired to correct the isobaric interference of 204Hg on 204Pb, so that the common Pb in the sample can be monitored. The relatively high background at mass 204, due to trace of Hg in the He gas, prevents small amounts of common Pb being detected, however. Remarkably, in the investigated samples the signal of 204(Pb+Hg) was always indistinguishable from the background and therefore no common Pb correction was applied. The 235U signal is calculated from 238U on the basis of the ratio 238U/235U = 137.818 (Hiess et al. Reference Hiess, Condon, McLean and Noble2012).
Laser-induced U–Pb fractionation and mass discrimination effects were simultaneously corrected using a matrix-matched external standard and considering the same integration intervals on the standard and the unknowns. Analyses were carried out using a spot size of 20 μm and a laser fluence of 8 J cm–2. The reference zircon GJ–1 (Jackson et al. Reference Jackson, Pearson, Griffin and Belousova2004) was adopted as external standard and the reference zircons 91500 (Wiedenbeck et al. Reference Wiedenbeck, Allé, Corfu, Griffin, Meier, Oberli, Von Quadt, Roddick and Spiegel1995) and 02123 (Ketchum et al. Reference Ketchum, Jackson, Culshaw and Barr2001) were selected as validation standards. Data reduction was carried out with the software package GLITTER® (van Achterbergh et al. Reference van Achterbergh, Ryan, Jackson, Griffin and Sylvester2001). Individual uncertainties given by the software for the isotope ratios were propagated relative to the respective reproducibility of the standard. This procedure was carried out for each analytical run as reported in Horstwood et al. (Reference Horstwood, Foster, Parrish, Noble and Nowell2003). ISOPLOT/Ex 3.00 software package by Ludwig (Reference Ludwig2003) was used for U–Pb apparent age calculations and representations. Data for external standards and validation standards are reported in online Supplementary Table S1 (available at http://journals.cambridge.org/geo).
5.b. Results
Zircon grains are generally prismatic and with dimensions up to 100 μm in length. They occur as porphyroclasts re-oriented parallel to S2 foliation. Zircon cores show mainly sector and broad banding zoning (Fig. 5d, g–l); homogeneous inner domains are also common (e.g. Fig. 5a–c, e–f). A peculiar feature, recognizable at the optical microscope (Fig. 3g) and well visible with CL imaging, is fringe-like tiny overgrowths elongated in the S2 HP–UHP foliation (Fig. 5a–d, g, j–l). Fringes are completely new portions of zircon without intergrowths with magmatic domains. Since the fringes are a few dozen microns in size, only those large enough to contain the spot were analysed. This selection avoided mixing rims with the inner (and older) cores, as confirmed by the consistency of the results and detailed in Figure 5, where the position of analyses is reported. Large zircons are frequently boudinaged (Fig. 3i); if zircon grains are separated into two or more fragments a narrow rim surrounds the different portions and is locally characterized by fringes parallel to S2 foliation, suggesting that boudinaging was coeval with the formation of these overgrowths (e.g. Fig. 5).

Figure 5. SEM images of zircon grains. (a–c) CL image of homogeneous zircon core with fringe-like syn-D2 overgrowth; (d) CL images of zircon grains showing sector and/or broad-banding zoning within cores and narrow rims with fringe-like syn-D2 overgrowth; (e, f) CL image of homogeneous zircon core with very few and tiny syn-S2 overgrowths; and (g–j, k–m) CL images of zircon grains showing sector and/or broad-banding zoning within cores and narrow rims with fringe-like syn-D2 overgrowth. Each image has the name of the zircon indicated and the large shaded circles indicate the spot analysed for U–Th–Pb geochronology, whereas smaller empty circles indicate spots analysed for trace elements. Letters differentiate circles in the same zircon, and correspond to the analyses in Tables 2 and 3. The concordant age of 168±8 Ma obtained for zircon 18-5 spot b is also indicated in (e).
Eleven zircon grains were selected for analysis and 23 U–Pb data were collected. Isotopic ratios (Table 3) define two main arrays on the Tera-Wasserburg diagram (Fig. 6a). According to Compston (Reference Compston1999) the lower intercept of the array should fix the crystallization age, whereas the upper intercept defines the common Pb composition. The major cluster is from zircon cores and yields a lower intercept age at 165±3.2 Ma (Fig. 6b). The minor cluster (four analyses) is from the tiny overgrowths and yields a lower intercept age at 65.5±5.6 Ma (Fig. 6c).
Table 3. Zircon isotopic data and apparent ages (zircon numbers refer to Fig. 5)


Figure 6. Tera-Wasserburg diagrams of isotopic ratios obtained from (a) all analysed zircon, (b) zircon cores and (c) syn-D2 overgrowths.
6. Pressure–temperature–deformation–time (P–T–d–t) evolution and discussion
Textural features, mineral compositions and U–Pb geochronology allow additional constrains to be imposed on the two evolution steps witnessed by the ZSZ serpentinites and detailed in Rebay, Spalla & Zanoni (Reference Rebay, Spalla and Zanoni2012).
Cores of the clinopyroxene and zircon porphyroclasts yield information on the protolith and more in general on the magmatic stage. The primary mineralogy of the rock is not preserved, so the identification of the rock type is not straightforward. Nonetheless, the negative Eu anomaly in both clinopyroxene and zircon (Fig. 4) suggests that the equilibrium melt already crystallized plagioclase. The abundant zircon and the relatively high concentrations of incompatible elements, such as Zr and Y, also suggest that the equilibrium melt was relatively evolved and not basaltic. Support for this conclusion is provided by the similar trace-element composition of analysed clinopyroxene as those from evolved lithologies of the Alpine Thetys such as the Fe–Ti oxide diorites (Tiepolo, Tribuzio & Vannucci, Reference Tiepolo, Tribuzio and Vannucci1997). Textural and chemical features of zircon and clinopyroxene coupled to the overall mineralogy of the rock (olivine/serpentine-dominated) suggest that the evolved melt likely percolated/intruded the lithospheric mantle. The occurrence of gabbro bodies and rodingite dykes nearby support this conclusion. Processes of melt percolation and melt-rock reaction between the oceanic lithospheric mantle and melts with MORB affinity are well documented in both the Lanzo Massif (Piccardo, Zanetti & Müntener, Reference Piccardo, Zanetti and Müntener2007; Piccardo et al. Reference Piccardo, Zanetti, Pruzzo and Padovano2007) and the Ligurian ophiolites (Sanfilippo, Tribuzio & Tiepolo, Reference Sanfilippo, Tribuzio and Tiepolo2014). U–Pb geochronology yields a date of 165±3.2 Ma for the zircon cores. Regarding mantle rocks, this date can either constrain the age of injection of the MORB-type melt into the mantle or the age of cooling of the oceanic lithosphere as a consequence of exhumation. Notwithstanding, the Middle Jurassic age is in perfect agreement with current knowledge on the age of formation of the Tethyan oceanic lithosphere in the Alps and Apennines (Rubatto, Gebauer & Fanning, Reference Rubatto, Gebauer and Fanning1998; Marotta et al. Reference Marotta, Roda, Conte and Spalla2016; Tribuzio et al. Reference Tribuzio, Garzetti, Corfu, Tiepolo and Renna2016).
Both clinopyroxene and zircon porphyroclasts have rims and fringes parallel to the S2 foliation at the strain cap and shadow, respectively, which crystallized at P–T conditions of 2.2–2.8 GPa and 580–620°C (Rebay, Spalla & Zanoni, Reference Rebay, Spalla and Zanoni2012). The new U–Pb data reveal that this HP–UHP event, registered by the recrystallization of zircon rims and fringes at these P–T conditions, likely occurred at 65.5±5.6 Ma and was followed by syn-D3 re-equilibration under epidote-amphibolite facies conditions (Fig. 7a; Rebay, Spalla & Zanoni, Reference Rebay, Spalla and Zanoni2012; Zanoni, Rebay & Spalla, Reference Zanoni, Rebay and Spalla2016). Radiometric ages obtained in this work extend the range 35–52 Ma reported in the literature for the Alpine HP–UHP re-equilibration towards older ages (Fig. 7b; Table 1). Remarkably, most of the literature ages have been obtained from samples that are within 20 km of the investigated area, and some are even nearer (≤5 km). Such geochronological heterogeneities imply that some sections of the ZSZ have likely experienced HP–UHP metamorphism earlier than previously thought; this supports the interpretation, based on contrasted P–T evolutions, that ZSZ is a complex containing different tectono-metamorphic units (e.g. Rebay, Spalla & Zanoni, Reference Rebay, Spalla and Zanoni2012; Weber & Bucher, Reference Weber and Bucher2015). The new ages are comparable to those already reported for the HP re-equilibration in the subducted continental crust of the Sesia-Lanzo Zone, which records the oldest subduction ages among the tectonic units of the Western Alps (Rubatto, Gebauer & Compagnoni, Reference Rubatto, Gebauer and Compagnoni1999; Handy & Oberhänsli, Reference Handy and Oberhänsli2004; Meda, Marotta & Spalla, Reference Meda, Marotta, Spalla, Spalla, Marotta and Gosso2010; Spalla et al. Reference Spalla, Gosso, Marotta, Zucali and Salvi2010; Cenki-Tok et al. Reference Cenki-Tok, Oliot, Rubatto, Berger, Engi, Janots, Thomsen, Manzotti, Regis, Spandler, Robyr and Goncalves2011; Rubatto et al. Reference Rubatto, Regis, Hermann, Boston, Engi, Beltrando and McAlpine2011; Roda, Spalla & Marotta, Reference Roda, Spalla and Marotta2012). Similar ages were also reported for the continental inliers of ZSZ (Beltrando, Rubatto & Manatschal, Reference Beltrando, Rubatto and Manatschal2010; Weber et al. Reference Weber, Sandmann, Miladinova, Fonseca, Froitzheim, Münker and Bucher2015; Fassmer et al. Reference Fassmer, Obermüller, Nagel, Kirst, Froitzheim, Sandmann, Miladinova, Fonseca and Münker2016).

Figure 7. (a) P–T–d–t evolution of the studied ZSZ rocks synthesized from Rebay, Spalla & Zanoni (Reference Rebay, Spalla and Zanoni2012) and Zanoni, Rebay & Spalla (Reference Zanoni, Rebay and Spalla2016). Metamorphic facies are after Spear (Reference Spear1993). GS – greenschist; BS – blueschist; EC – eclogite; EA – epidote-amphibolite; AM – amphibolite; GR – granulite. Geotherms after Cloos (Reference Cloos1993): (1) near spreading ridge or volcanic arc; (2) normal gradient of old plate interior; (3a) warm subduction zones; and (3b) cold subduction zones. (b) Tectonic sketch map of the northwestern Alps with the location of dated samples in the ZSZ. The inset shows the Western Alps with location of dated samples at Monviso. Nappe systems: Penninic continental nappe (MR – Monte Rosa; AB – Arcesa-Brusson; GP – Gran Paradiso; SB – Gran St Bernard); Austroalpine nappe (DB – Dent Blanche; MM – Mont Mary; P – Pillonet; AR – Acque Rosse; CH – Chatillon-St Vincent; E – Etirol-Levaz; G – Grun; EM – Mt Emilius; GR – Glacier-Refray; S – Santanel; TP – Tour Ponton; SL – Sesia-Lanzo Zone; Vp – Valpelline series; Dk – Diorite-kinzigitic series, Ar – Arolla series; Gm1 – Gneiss Minuti Complex, non-eclogitic; Gm2 – Gneiss Minuti Complex, with eclogitic relicts; Emc – Eclogitic Micashist Complex; Mesozoic metasedimentary cover (black): R – Roisan Zone); and Ophiolite Piedmont Zone (CO – Combin Zone; PCB – Pancherot-Cime Bianche; FC – Faisceau de Cogne; ZS – Zermatt-Saas; MA – Mt Avic; A – Antrona ophiolite). Tectonic lines: CL – Canavese tectonic line; AR – Aosta-Ranzola fault system; SF – Simplon normal fault; MD – Mont Dolent; B – Biella; M – Miagliano; SC – Redrawn after Dal Piaz (1999).
Older ages interpreted as dating the P–T prograde evolution (Table 1; Skora et al. Reference Skora, Lapen, Baumgartner, Johnson, Hellebrand and Mahlen2009, Reference Skora, Mahlen, Johnson, Baumgartner, Lapen, Beard and Szilvagyi2015) indicate that the subduction of ZSZ oceanic slices was already active at 80 Ma. Our data are therefore coherent with oceanic lithosphere subduction, necessary to justify the low thermal regime under which Western Alpine continental crust was exhumed (e.g. Zucali, Spalla & Gosso, Reference Zucali, Spalla and Gosso2002; Roda, Spalla & Marotta, Reference Roda, Spalla and Marotta2012).
Scattered peak ages (41–72 Ma; Cliff, Barnicoat & Inger, Reference Cliff, Barnicoat and Inger1998; Table 1) are also observed in the Monviso Massif, supporting the interpretation that it represents a complex of ophiolitic HP slices accreted during the final stages of their exhumation (Schwartz et al. Reference Schwartz, Lardeaux, Guillot and Tricart2000; Guillot et al. Reference Guillot, Schwartz, Hattori, Auzende and Lardeaux2004). The detection of contrasted petrologic and chronologic histories in both the ZSZ and Monviso Massif confirms that the HP metaophiolites of Piedmont zone is a highly heterogeneous domain composed of slices of variable size, from one to tens of kilometres3 (e.g. Guillot et al. Reference Guillot, Schwartz, Hattori, Auzende and Lardeaux2004).
7. Conclusions
In summary, our results allow us to demonstrate that multiscale structural analysis assisted by detailed thermodynamic modelling is a fundamental tool for dating single deformation stages and associated mineral transformations.
The results confirm a Middle Jurassic age for oceanic crust accretion, which is in agreement with that observed in the ophiolitic complexes from the Alps and Apennines.
Finally, our results allow us to widen the time span under which rocks of ZSZ recorded HP–UHP conditions during 70–38 Ma and to infer that syn-D2 eclogite facies metamorphic imprint developed at 65.5±5.6 Ma under a very low thermal regime, as suggested by the relationships with geotherm inferred by Cloos (Reference Cloos1993) for cold subduction zones (Fig. 7). These findings go along with the cold thermal regime under which the Sesia-Lanzo Zone was exhumed, before intrusion of the Periadriatic magmas, which is compatible with ongoing oceanic subduction (e.g. Zucali, Spalla & Gosso, Reference Zucali, Spalla and Gosso2002; Zanoni, Spalla & Gosso, Reference Zanoni, Spalla and Gosso2010; Roda, Spalla & Marotta, Reference Roda, Spalla and Marotta2012; Roda & Zanoni, Reference Roda and Zanoni2016).
Heterogeneous HP–UHP ages and contrasted P–T–d–t evolution within ZSZ can be explained either by: considering slices of ophiolites within a hydrated mantle wedge that follow the same path at different times in a subduction system, where they can be tectonically sampled at different depths; or considering that they can follow different paths. These ophiolite slices can be coupled and/or decoupled at different times in a relatively long-lasting subduction system where oceanic and continental lithosphere are involved, as is the case for the Western Alps.
Declaration of interest
No conflict of interest exists for the authors.
Acknowledgements
G.R., D.Z. and M.I.S. acknowledge funding from MIUR (Ministero dell'Istruzione, dell'Università e della Ricerca) PRIN 2010–2011 (grant number 2010AZR98L) ‘Birth and death of oceanic basins: geodynamic processes from rifting to continental collision in Mediterranean and circum-Mediterranean orogens’ and G. Gosso, A.M. Marotta and M. Zucali for fruitful discussions on Alpine dynamics. D.Z. and P.L. acknowledge funding from the projects ‘Deformazione e metamorfismo delle ofioliti di alta pressione nelle Alpi Occidentali’, Università di Pavia and ‘Analisi delle impronte strutturali e metamorfiche delle rocce femiche e ultrafemiche della Zona Zermatt-Saas (alta Valtournanche) per la ricostruzione della loro evoluzione geodinamica’, Università di Milano, respectively. We wish to thank Gaetano Ortolano, Giovanni Capponi and an anonymous reviewer for useful suggestions and discussions that improved the manuscript.
Supplementary material
To view supplementary material for this article, please visit http://doi.org/10.1017/S0016756817000334