The editors of Microscopy Today congratulate the 10 winners of the 11th Microscopy Today Innovation Awards competition. The innovations described below advance microscopy in the areas of light microscopy, electron microscopy, and microanalysis. These innovations will make microscopy and microanalysis more powerful, more productive, and easier to accomplish.
Bond-Selective Transient Phase Microscope
Boston University
Developers: Delong Zhang, Lu Lan, Gabriel Popescu, and Ji-Xin Cheng

In the widely used phase contrast microscope, the optical phase of photons passing through a sample is largely insensitive to the chemical composition inside, making it difficult to investigate the molecular interactions in complex biological and material systems. The new Bond-Selective Transient Phase (BSTP) microscope solves this long-standing bottleneck by probing the transient phase change resulting from the molecular vibrational absorption of a pulsed mid-infrared (IR) excitation.
The BSTP microscope operates in the following manner. First, a diffraction phase microscope is used to acquire a quantitative phase image of an unperturbed sample, a “cold” frame. Next, mid-IR pulses illuminate the sample, generating absorption and a local temperature rise, which transiently modifies the local refractive index and the quantitative phase image, a “hot” frame. However, the temperature rise lasts only a few microseconds, and thus the phase change cannot be captured with current recording devices operating at only a few thousand frames per second. To achieve sub-microsecond temporal resolution, a time-gated phase imaging scheme captures the phase change resulting from a mid-IR pulse that produces the “hot” frame. Finally, by comparing the “hot” and “cold” phase images, a differential phase image is obtained without the high background signal from water in the sample. This differential phase image reveals characteristic molecular vibrational effects at chemical bonds. By sweeping the excitation wavelength of mid-IR pulses, a hyperspectral image stack is acquired to reveal molecular information inside the samples.
Previously, phase imaging has been combined with fluorescent labeling to provide molecular specificity. However, fluorescent labels have fundamental limitations, including photo-bleaching, perturbation of biological structures, and inability to label small molecules. The BSTP microscope provides molecular information in phase microscopy without the need for labels. In one example, BSTP imaging showed that features of a living cell involved in CH2 asymmetrical stretching (2930 cm−1) could be distinguished from features involved in CH3 stretching (2950 cm−1). Live cell BSTP imaging can be obtained at a 50 Hz frame rate with high spectral fidelity, sub-microsecond temporal resolution, and sub-micron spatial resolution. Applications envisioned include single-cell biochemistry, cell-drug interactions, and potentially the discovery of biomarkers that lead to diagnosis and treatment.
RCM-NIR Microscope Upgrade
Confocal.nl
Developer: Erik Manders
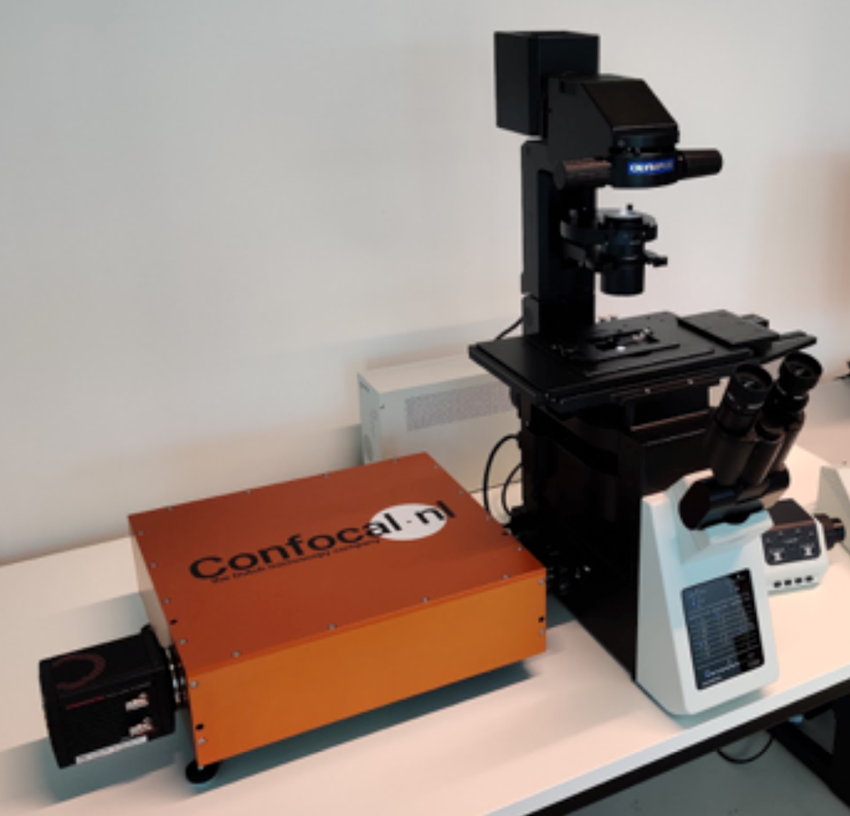
The RCM-NIR module is a microscope upgrade allowing super-resolution confocal 3D imaging in the near-infrared (NIR) light spectrum (800–1000 nm). The RCM-NIR allows penetration up to 1 mm deep into the specimen without the need for any specialized equipment other than a conventional microscope setup with a low-power (non-damaging) laser. The imaging setup consists of an RCM-NIR upgrade module, a fluorescence microscope, a CMOS camera, a laser combiner (785 nm and 640 nm excitation at 20 mW), and broad-spectrum LED illumination.
The RCM-NIR uses Confocal.nl re-scan technology as a basis. As in a standard confocal microscope, the scanning unit scans the laser light in the sample and de-scans the emission light, directing it at the pinhole. After the pinhole, a second re-scan unit directs the light onto a camera chip. During imaging, the re-scan mirrors move with a larger amplitude than the first scan mirrors. This magnifies the image on the camera chip compared to the sample and eventually results in the higher resolution of the image. By using a sensitive camera as detector, the signal-to-noise ratio of the RCM is 4 times higher than in standard confocal microscopy. The camera-based detection scheme makes collection of the NIR-spectrum possible; whereas, the PMTs used in conventional confocal microscopy are not sensitive to NIR photons. Imaging with the RCM-NIR module improves resolution by a factor of √2 (approximately 40%) compared to Abbe's resolution limit. As an example, upon excitation at 785 nm, the optical resolution achieved by traditional imaging is 360 nm, whereas with the RCM-NIR the resolution is improved to 260 nm, nearly identical to the resolution achieved with green wavelengths on a regular confocal microscope.
NIR wavelengths penetrate deeper into tissue and materials, enabling examination of thicker specimens. Exposure to NIR wavelengths is far less damaging to cells compared to light in the visible spectrum, a benefit for live cell imaging. Also, because of the low absorbance and scattering in biological tissue, imaging with NIR wavelengths results in an image with high contrast and sensitivity. Since NIR wavelengths are employed in image-guided surgeries, the RCM-NIR module makes on-site/on-line pathology in the operating room possible.
Stream System
DENSsolutions B.V.
Developers: Hugo Pérez, Hongyu Sun, Mathilde Lemang, Anne Beker, and Tijn van Omme

The “Stream” System uses an innovative micro-electromechanical system (MEMS) device to accurately control the liquid environment inside the TEM. This device, referred to as the Nano-Cell, consists of a dual-cell chip technology to form a miniature reaction chamber at the tip of a side-entry TEM specimen holder. The bottom chip contains an inlet and outlet surrounded by thin spacers that define the height of the liquid chamber. The top chip sits on the upper surface of the spacers and confines the liquid inside a well-defined microfluidic channel that brings the liquid specimen under the electron beam. The chips are sealed with an O-ring, which enables the liquid to stay within the cell. Since both the inlet and outlet within the Nano-Cell can be individually pressurized (for example, positive pressure on the inlet and negative pressure on the outlet), the user can accurately control the pressure-driven flow of the liquid without bypassing the region of interest. This configuration also controls the bulging of the membranes, which therefore enables the user to significantly control the liquid thickness. Nano-Cells have been designed to provide biasing with three electrodes (working, reference, and counter electrodes) useful for electrochemistry experiments. The Nano-Cell also has microheaters for control of the temperature up to 100°C. Elemental analysis can be by energy-dispersive X-ray emission spectrometry (XEDS).
The Stream System overcomes several difficulties typical of liquid-phase TEM experiments. It provides controlled flow through the region of interest, and it reduces beam broadening effects by reducing the liquid layer thickness, reducing the background intensity for electron diffraction of liquids. With control over pressure and flow, unwanted beam-induced species can be flushed away, and bubbles can be flushed or fully dissolved.
The Stream System can be applied to materials synthesis for the study of nucleation and growth, as well as chemical and electrochemical reactions. In research on energy devices, liquid phase microscopy is important for development of advanced electrodes and electrocatalysts in devices such as batteries, supercapacitors, and fuel-cells. In life sciences it is now possible to image whole biological cells in liquid, resolve fine structure in biomaterials and proteins in their native liquid state, and study biological dynamics in real time.
Stroboscopic Ultra-Fast Electron Microscopy
Euclid Techlabs, NIST, and Brookhaven National Lab
Developers: Chunguang Jing, Alexei Kanareykin, Eric Montgomery, Yubin Zhao, Wade Rush, Ao Liu, Ilya Ponomarev, Yimei Zhu, and June Lau

A relatively inexpensive pulsing device converts an existing transmission electron microscope (TEM) into an ultrafast time-resolved TEM (UTEM). The electron beam produced in a conventional TEM is continuous (dc), so imaging and diffraction are accomplished in a static time-integrated manner. Now it is possible to unite the time domain with the spatial domain to create four-dimensional electron microscopy. Euclid's innovation is to use RF technology to manipulate the TEM electron beam, using methods developed for electron beam accelerators, to produce a fundamentally different stroboscopic instrument. The pulser device can be built on any TEM because the electron emission process is unchanged. This pulsing device consists of a series of magnetic quadrupoles and stripline resonators designed to achieve broad tunability not possible with deflecting cavities. This apparatus modulates and chops the incoming dc electron beam and converts it into pico- and sub-picosecond (100 fs to 10 ps) electron pulses with a large range of repetition rates from 1 Hz to 12 GHz. This modification requires no change in the complex electron optics that make up the core of a TEM.
Compared to a time-resolved TEM relying on a sophisticated and expensive pump-probe femtosecond laser system, no laser is required. In addition, the RF pulser technology allows extended ranges of repetition rates and duty cycle tunability, which are not achievable in a laser-based UTEM. Compared to existing commercial systems, a lower price and retrofit compatibility make UTEM affordable for a broader scientific community. The Euclid RF pulser can be retrofitted to old TEMs or pre-installed in new TEMs. The keen interest from a world-leading manufacturer of TEMs confirms the significance of the technology. Currently these pulsers are operational at the US National Institute of Standards and Technology and at Brookhaven National Laboratory.
Applications include development of devices based on advanced functional materials: electronic (transistors, communication systems), ionic (batteries, supercapacitors, memristors), magnetic (spintronics, magnetic memory), photonic (lasers), and electromechanical (NEMS/MEMS) materials and their hybrids. Recently discovered radiation damage mitigation using the Euclid stroboscopic technique should make this type of UTEM beneficial for biological and biomedical research, in particular, for cryo-EM studies of bio samples.
CX-A: Live Cell Imaging in Multi-Well Plates
Nanolive SA
Developer: Sebastien Equis

The Nanolive CX-A microscope automates image data acquisition through an intuitive user interface that enables first-time users to set up experiments in just a few minutes and walk away, while the CX-A automatically collects the images. In addition to improving data significance, multiple imaging regimens can be programmed within the same multi-well plate, allowing users to run different applications in parallel. A real-time preview allows the user to navigate through the data at any time. Living cells can be imaged for long periods of time. Thanks to its harmless way of cell preparation and observation, hundreds of images can be collected every hour, transforming endpoint assays into continuous analysis. Experiments can last for days or weeks at a time, while cells remain unperturbed in a physiologically controlled environment.
From the hardware point of view, the CX-A is the first and only holo-tomographic microscope compatible with multi-well plates that can image living cells for extended times without sacrifices in resolution. The large field of view (up to 1 mm × 1 mm) of the CX-A allows monitoring of cells at the population level, in multiple dishes, and in parallel. The Nanolive imaging technology (non-invasive, label-free, three-dimensional, high-resolution) provides contrast on live specimens with zero phototoxicity and zero photobleaching. The combination of this imaging technology with the new CX-A delivers a walk-away solution for long-term live cell imaging of cell organelles, single cells, and cell populations. This next-generation microscope allows observation of living cell populations all the way down to individual organelles with a resolution of < 200 nm. Compatibility with multi-well plates (for example, 96-well plates) allows scientists to test multiple conditions in parallel, bringing statistical significance to every experiment.
Results from such studies can provide insights into how cells interact, how organelle morphologies change, and how organelles interact. Long-term imaging allows researchers to visualize biological processes unfolding in real time at high spatial and temporal resolution. For example, the CX-A allows users in the pharmaceutical industry to perform multiple label-free drug perturbation assays, live and over long periods of time. Such capabilities can enhance drug screening, target identification, and mechanism of action studies.
Accelerating Iterative Deconvolution and Multiview Fusion
National Institute of Biomedical Imaging and Bioengineering
Developers: Min Guo, Yicong Wu, and Hari Shroff

It is now possible to accumulate massive amounts of light microscopy data with the click of a button—in a single day a light-sheet microscope can generate hundreds of terabytes of data. This capability has led to a “data deluge” that impedes scientific discovery. Deconvolution is an important post-acquisition step; however, this can take much longer than data collection. Stitching and registration are even more time-consuming image processing operations. Algorithms and software have been created for drastically reducing the time required for deconvolving, stitching, and registering large light microscopy datasets. These innovations permit up to several thousand-fold faster deconvolution and multi-view fusion than previous software.
A number of software improvements were made to achieve these speeds. First, methods were adapted from medical imaging, where an unmatched back projector accelerates Richardson-Lucy deconvolution by at least 10-fold, in most cases requiring only a single iteration. Second, improvements in 3D image-based registration and stitching with a graphics processing unit (GPU) resulted in increased speeds of 10-fold to 100-fold over CPU processing. This performance advantage is important given that registration and stitching are more time-consuming than deconvolution. Third, deep learning can provide further accelerations, particularly for deconvolution with a spatially varying point spread function. A novel neural network architecture, “DenseDeconNet,” was created for this purpose, and the unmatched back projector method was used to train the neural network. These methods are effective on diverse samples including single cells, nematode and zebrafish embryos, and cleared mouse tissue. Finally, these methods facilitate the use of new computational imaging microscopes that would be difficult to operate without these improvements in data processing speed. The methods described here are freely available as open-source software written in the MATLAB, CUDA, and Python programming languages.
Two microscopy companies and ten labs across the world have tested these algorithms on their microscopy data, and they report similar speed-up factors. Even though these methods are only a year old, there is strong reason to suspect that any light microscopy dataset that requires deconvolution, image registration (for example, multiview light-sheet microscopy), or stitching is likely to enjoy massive acceleration in the rate of these post-processing methods.
Isotope-Resolved Electron Energy-Loss Spectroscopy
Oak Ridge National Laboratory, University of Illinois at Chicago, and Nion Co.
Developers: Juan-Carlos Idrobo, Jordan Hachtel, Robert F. Klie, Jacob R. Jokisaari, and Ondrej Krivanek

The identification of isotopes is often accomplished using destructive characterization approaches, such as mass spectrometry. Alternatively, isotopes can be resolved through shifts in the vibrational modes using infrared or Raman spectroscopy, as well as neutron scattering. However, these characterization approaches all lack the spatial resolution to identify the location or dynamics of the isotopes and, moreover, require relatively large quantities of sample for accurate measurements. Improvements in spatial sensitivity have been achieved using tip-enhanced Raman spectroscopy (TERS) and scanning near-field optical microscopy (SNOM) to examine the vibrational spectra of biomolecules with spatial resolutions of 10 to 25 nm.
Recent electron monochromators on aberration-corrected STEM instruments have allowed electron energy-loss spectroscopy (EELS) to access vibrational modes in solids with spatial resolutions down to 0.2 nm. When the energy spread of the electron beam is limited to 10 meV or less, the spectral resolution is sufficient to resolve vibration peaks in molecules. Even different isotopes of the same substance can be resolved by this method (Isotope Resolved-EELS). To examine vibrational peaks in a liquid, two layers of hexagonal boron nitride were configured to encapsulate the liquid. The liquid cell was prepared by growing BN films using chemical vapor deposition. Liquid water was sealed between two BN layers by Van der Waals interactions and the surface tension of the water. Examination of a water-deuterium mixture at 60 keV in such a wet cell allowed the O-D stretch peak (310 meV loss, 2500 cm−1) to be clearly distinguished from the O-H stretch peak (421 meV loss, 3400 cm−1). As expected, the heavier isotope is observed at a lower energy loss. The signal from deuterium is weak; thus, the experiment above required the average of 100 spectra and careful subtraction of the background. Other experiments have revealed 13C labels in L-alanine with atomic-site resolution.
When combined with cryogenic sample preparation or liquid cells consisting of ultra-thin graphene or BN window-layers, isotope-labeled ions or molecules can be tracked in battery cathodes, solid oxide-fuel cell membranes, or biological cells with the spatial resolution of a transmission electron microscope. This capability enables direct observation of intracellular molecular chemistry.
Label-Free Super-Resolution Localization Photoacoustic Microscopy
Pohang University of Science and Technology and Opticho Inc.
Developers: Jongbeom Kim, Jin Young Kim, and Chulhong Kim

In photoacoustic microscopy (PAM) an image is reconstructed from ultrasonic waves induced by a transient thermal expansion of molecules receiving a short pulse of laser light. The physical characteristics and arrangement of the laser, mirror scanner, and the acoustic detector determine the attainable signal-to-noise ratio (SNR), spatial resolution, and temporal resolution. Existing systems may have high SNR and spatial resolution but operate rather slowly.
Label-free super-resolution localization PAM employing a galvanometer scanner (L-PAM-GS) delivers a high-quality photoacoustic image at a faster rate than previously available PAM systems. A nanosecond pulsed laser with a pulse repetition rate of 600 kHz at a wavelength of 532 nm was used to enable ultra-fast, high-contrast vascular imaging capabilities. Using a ring transducer that allows the laser beam to pass through a hole in its center, the ultrasound or laser beam is aligned to maximize a signal-to-noise ratio (SNR). The galvanometer scanner is installed vertically, submerging only the mirror part of the scanner in the water. This submerged mirror part steers both the laser beams and the photoacoustic waves underwater in a coaxial and confocal formation, leading to a fast imaging speed, a high SNR, and a wide scanning range. This configuration allows application of a localization process to in vivo PAM images without a contrast agent. This method locally highlights the PA signals generated from red blood cells (RBC), momentarily captured during the PA imaging experiments, leading to high resolution with a low optical NA.
Improvements with the L-PAM-GS allow noninvasive observations of microvasculature in small animals and humans in vivo. Further, functional hemodynamics, the blood flow rate in microvasculature, was successfully monitored and quantified in vivo. By localizing photoacoustic signals from RBCs without contrast agent, unresolved microvessels were clearly distinguished, and the spatial resolution in vivo was improved by a factor of 2.5. The L-PAM-GS device can potentially be useful for noninvasively delineating stroke lesions in vivo. The label-free localization method provides super-resolved images from blood vessels under flow conditions, but not from blocked blood vessels. If a blood vessel is blocked and RBCs are not flowing, such as in strokes, the blood vessel is not visible.
PrismaXRM
Sigray, Inc.
Developer: Wenbing Yun

Three-dimensional X-ray microscopy (also known as micro-CT) has developed substantially in the past two decades, and many systems are now reaching the limits of resolution achievable at high throughput. PrismaXRM was designed to overcome this resolution limit through a novel, multi-contrast approach that provides critical information on sub-resolution features, such as cracks and nanoparticles. The PrismaXRM system takes advantage of the fact that X-rays interact with samples through a variety of means. Until now, X-ray imaging systems, including medical X-ray units and X-ray microscopes, have relied on absorption contrast to produce images. However, X-rays are also slightly refracted from their path, resulting in a phase shift of the X-ray waves transmitted through the object and scattered at an angle. Such phenomena have been challenging to detect until the recent innovation of grating-based interferometry (GBI).
PrismaXRM employs a grating-based approach to enable simultaneous acquisition of three modes of contrast: absorption, Quantitative Phase™, and Subresolution Darkfield™, that have proven to be powerful in their complementary nature. A key enabling feature of the system is Sigray's microstructured X-ray source. The source employs a diamond target with an array of embedded microstructures to provide the illumination required for the grating-based technique to work. When the structured illumination is incident upon a downstream grating (G1), a pattern of constructive and destructive interference fringes is produced. Measurement of how a sample alters the interference fringe field through absorption (attenuation of fringe), phase (shifting of the fringe), and darkfield (amplitude decrease) provides the simultaneous acquisition of the three contrast mechanisms. The three modes of contrast complement each other by providing information that the others may not detect. Quantitative Phase™ enables determination of material refractive indices and distinguishes between similarly absorbing materials such as two types of polymers. Subresolution Darkfield™ provides access to information on features below the limitations of resolution, such as nanoparticles, pores, and cracks.
Applications range from semiconductor devices to lithium batteries to carbon fiber-reinforced polymers to concrete, including in situ observations of low-contrast fluid flow through pore networks. For example, Subresolution Darkfield™ has elucidated patterns of lithium ion nanoparticle migration into a porous carbon cathode in an intact lithium-air battery.
ORION NanoFab SIMS
Carl Zeiss SMT
Developers: Sybren Sijbrandij, David Dowsett, and Tom Wirtz

The ORION NanoFab uses a gas field ionization source (GFIS) to produce a well-focused beam of helium or neon ions with remarkably small probe sizes, typically 0.5 nm and 1.9 nm, respectively. This instrument provides high-resolution helium ion microscopy (HIM), focused ion beam (FIB) milling, and now microanalysis via secondary ion mass spectrometry (SIMS). The optional SIMS spectrometer is fully retractable, so it does not interfere with any of the instrument's other capabilities when not in use. As a focused neon beam sputters the sample, those charged atoms, molecular fragments, and small clusters are extracted and directed to the spectrometer. Positive or negative species can be collected with the polarity switchover taking less than a minute. Under the influence of a magnetic field, the charged particles are sorted based on their mass-to-charge ratios as they are individually focused to the detector focal plane. There are five channeltron detectors: one for measuring the total ion current and four that can be positioned to collect the signal corresponding to a specific mass-to-charge ratio. The range of detectable masses spans from hydrogen to uranium with a mass resolution of m/Δm ~ 400.
Thus equipped, the NanoFab can serve many applications with a variety of workflows. Most commonly, the operator will first use the 0.5 nm helium ion beam to navigate and save images of the features of interest before switching to neon ions to begin the SIMS analysis. If desired, a full mass spectrum can be acquired by sweeping the magnetic field or moving the detectors. When the magnetic field strength is held constant, the detectors may be moved to collect the masses of interest.
Scanning the neon beam in a traditional raster pattern allows mass-filtered images to be acquired with a spatial resolution of about 10 nm, revealing distributions of chosen atoms, molecular fragments, or clusters. Repeated rastering of the same area will progressively expose deeper layers, and the sequence of images can be combined to reveal 3D compositional information. Unlike SEM with EDX, this technique is extremely surface sensitive, can distinguish different isotopes, and needs no special provisions to see light elements like lithium or hydrogen.