The birth of insect pathology in Canada
Diseases of insects have been noted and studied for millennia. Aristotle’s description of honey bee (Apis mellifera Linnaeus; Hymenoptera: Apidae) diseases in ~300 BCE is often viewed as the beginning of the recorded history of insect pathology. Basic knowledge acquired over centuries by observing infectious diseases in silk worms (Bombyx mori Linnaeus (Lepidoptera: Bombycidae) and honey bees culminated by the end of the 19th century in an emerging interest to not only suppress pathogens in beneficial insects but also in their use to control pest insects (Steinhaus Reference Steinhaus1975). It was not until the middle of the 20th century that the study of insect pathogens came into its own as a recognised and unified discipline (e.g., Steinhaus Reference Steinhaus1945, Reference Steinhaus1949).
In Canada, insect pathology was a neglected area of research until after the Second World War. The potential of insect diseases to control forest insects was recognised early on by J.J. de Gryse, who was director of the Agriculture Department’s Division of Forest Insects, Ottawa, Ontario, Canada between 1934 and 1952. In that capacity, he was instrumental in organising the basic structure underlying today’s federal government research in forest entomology, including establishment of the Forest Insect Survey (1936) and the Forest Insect Laboratory in Sault Ste. Marie, Ontario, Canada (1940) (Wallace Reference Wallace1990). De Gryse advocated for an insect diseases research programme as early as 1923 (Steinhaus Reference Steinhaus1975). His vision did not become reality until the late 1940s when the government allocated CDN$150 000 to build a laboratory for the study of forest insect pathogens in Sault Ste. Marie. By the time the Laboratory of Insect Pathology had been completed (1950, Fig. 1), the price tag approached CDN$750 000, the equivalent of roughly CDN$7.0 million today.

Fig. 1 The Laboratory of Insect Pathology in Sault Ste. Marie in the early 1950s. The Laboratory was renamed the Insect Pathology Research Institute in 1959. This institute was merged with the Chemical Control Research Institute in 1977 to form the Forest Pest Management Institute, which merged with the Ontario Regional Forestry Centre in 1997 to form the current Great Lakes Forestry Centre. Photograph credit: Canadian Forest Service.
The Canadian government’s decision to fund the new facility was swayed by a fortuitous biological control success earlier that decade which demonstrated the promise of insect pathogens. An outbreak of the European spruce sawfly, Gilpinia hercyniae (Hartig) (Hymenoptera: Diprionidae) in eastern Canada during the 1930s led to the introduction of parasitoids from Europe (Reeks Reference Reeks1953). However, collapse of the outbreak between 1938 and 1942 was attributed largely to a nucleopolyhedrovirus, which likely came from Europe with the parasitoid material (Balch and Bird Reference Balch and Bird1944; Bird and Elgee Reference Bird and Elgee1957). The combined action of virus and parasitoids is credited for keeping spruce sawfly populations at endemic levels (Neilson and Morris Reference Neilson and Morris1964). That success, bolstered by a rapidly expanding outbreak of the spruce budworm, Choristoneura fumiferana (Clemens) (Lepidoptera: Tortricidae) in eastern Canada, helped de Gryse persuade the government that a laboratory for the study of insect pathogens was a necessary addition to the federal government’s forest insect research capacity in Sault Ste. Marie (Steinhaus Reference Steinhaus1975). Around the same time, the federal government established the Laurentian Forestry Centre in Québec, Québec, Canada (1952), which also embraced an active research programme in forest insect pathology (Cloutier et al. Reference Cloutier, Perron and Jean2008). Forest pest management thus became the main theatre for the development and practice of insect pathology in Canada during the second half of the 20th century.
The goal of the federal forest insect pathology research programme was articulated by a visionary de Gryse in personal correspondence to Steinhaus in 1949: “I hope that, before long, we may achieve some practical results, but I am far more interested in the immediate developments of fundamental research. The rest will come in its own good time and will be more assured of success if the work is performed on a scientific basis” (Steinhaus Reference Steinhaus1975). Both objectives would be realised over the ensuing 65 years, as Canadian researchers in government and university laboratories, in close collaboration with insect pathologists in the United States of America and elsewhere, made numerous contributions to our understanding of forest insect pathogens and how they can be used to control forest insect pests. Those contributions, culminating in the first large-scale commercial use of a microbial pesticide in the world, will be reviewed in this paper. What follows is a more or less chronological overview of main research thrusts and developments and key outcomes in sections arranged by conventional major pathogen divisions (Fungi, Microsporidia, Viruses, and Bacteria) and supported by a necessarily limited selection of the plethora of publications related to forest insect diseases that have been published since the birth of forest insect pathology in Canada.
Fungi
Knowledge of fungal entomopathogens was still in its infancy when forest insect pathology started to take shape in Canada. Although work in the late 18th century by Metchinikoff (in the Ukraine) and Snow (in Kansas) had firmly established the potential of fungi for biological control of agricultural pests (Lord Reference Lord2005), little was known about fungi infecting forest insects. Research by government scientists focussed on the acquisition of foundational knowledge on occurrence (Smirnoff and Jobin Reference Smirnoff and Jobin1973; Smirnoff and McLeod Reference Smirnoff and McLeod1973), taxonomy (MacLeod Reference MacLeod1956; Tyrrell Reference Tyrrell1972), morphology (MacLeod et al. Reference MacLeod, Müller-Kögler and Wilding1976), biochemistry (Brennan et al. Reference Brennan, Griffin, Lösel and Tyrrell1975), and natural history (Tyrrell and MacLeod Reference Tyrrell and MacLeod1972; Tyrrell Reference Tyrrell1977; Remaudière et al. Reference Remaudière, Latgé and Smirnoff1978) of fungi infecting forest insects. There was a predominant interest in Entomophthorales, a group of fungi that stood out in their ability to cause local or regional epizootics in arboreal insect populations, in particular species infecting spruce budworm (Vandenberg and Soper Reference Vandenberg and Soper1978); eastern hemlock looper, Lambdina fiscellaria fiscellaria (Guenée) (Lepidoptera: Geometridae) (Otvos et al. Reference Otvos, MacLeod and Tyrrell1973; Smirnoff and Jobin Reference Smirnoff and Jobin1973); and forest tent caterpillar, Malacosoma disstria Hübner (Lepidoptera: Lasiocampidae) (MacLeod and Tyrrell Reference MacLeod and Tyrrell1979). The federal research effort was complemented by decades of collaborative research on the same host-pathogen systems at McGill University (Montréal, Québec, Canada) and Memorial University (St. John’s, Newfoundland and Labrador, Canada). That work included studies on cellular immune response in forest insects (Dunphy and Nolan Reference Dunphy and Nolan1980, Reference Dunphy and Nolan1981, Reference Dunphy and Nolan1982a), comparative development and physiology (Dunphy et al. Reference Dunphy, Nolan and MacLeod1978), and influence of culture media and physical factors on germination, growth, morphogenesis, and mycotoxin production (Nolan and Dunphy Reference Nolan and Dunphy1978; Dunphy and Nolan Reference Dunphy and Nolan1982b; Dunphy et al. Reference Dunphy, Chadwick and Nolan1985).
Much of this research was motivated by field observations that entomophthoralean mycosis can affect over 95% of local insect populations (Perry Reference Perry1985; Perry and Régnière Reference Perry and Régnière1986). Manipulation for effective biological control proved to be an elusive target, however. Efforts to initiate epizootics in a spruce budworm outbreak in Newfoundland by inoculative release of larvae infected with Entomophthora egressa MacLeod and Tyrrell (Zygomycota: Entomophthorales) and Zoophtora radicans (Brefeld) Humber, Ben Ze’ev, and Kenneth (Zygomycota: Entomophthorales) did not succeed (Lim et al. Reference Lim, Raske, Tyrrell and Perry1981; Lim and Perry Reference Lim and Perry1983). Subsequent inundative releases in the United States of America with mist blower applications of mass-produced Z. radicans hyphal bodies failed to increase prevalence of infection (Soper Reference Soper1985). Failures of field applications emphasised the need to better understand the complexity of processes underlying sporulation and germination of infectious stages (Perry and Fleming Reference Perry and Fleming1989a), dormancy and persistence of infectivity (Perry Reference Perry1982), and timing of infection (Perry and Fleming Reference Perry and Fleming1989b) relative to phenology, movement and density of the target pest (Perry and Régnière Reference Perry and Régnière1986; Perry et al. Reference Perry, Tyrrell and Strongman1995), as well as effects of weather conditions on those interactions. Work was initiated to develop simulation models to optimise introduction of fungal inocula into forest insect pest populations (Fleming and Perry Reference Fleming and Perry1986), but was discontinued when the principal investigator (D.F. Perry) left the Canadian Forest Service.
Interest in Zoophthora and Entomophthora was superseded during the 1990s by a focus on Entomophaga aulicae (Reichardt in Bail) Humber (Zygomycota: Entomophthorales), a species long known to cause dramatic local epizootics in outbreaks of defoliating forest Lepidoptera (Perry and Régnière Reference Perry and Régnière1986; Perry et al. Reference Perry, Tyrrell and Strongman1995). Research addressed conditions controlling production of conidia (McDonald and Nolan Reference McDonald and Nolan1995), protoplasts and hyphal bodies (Nolan Reference Nolan1988, Reference Nolan1993), and its mode of action and pathogenesis in forest insects (Tyrrell Reference Tyrrell1990; Milne et al. Reference Milne, Wright, Welton, Budau, Gringorten and Tyrrell1994). Interest in Entomophaga was reinforced by the discovery of E. maimaiga Humber, Shimazu, and Soper (Zygomycota: Entomophthorales) in outbreaks of gypsy moth, Lymantria dispar (Linnaeus) (Lepidoptera: Erebidae) in 1989 and its subsequent rapid spread throughout eastern North America (Elkinton et al. Reference Elkinton, Hajek, Boettner and Simons1991). Researchers at the University of Toronto (Toronto, Ontario, Canada) developed molecular methods for unequivocal identification (Walsh et al. Reference Walsh, Tyrrell, Humber and Silver1990), which enabled confirmation of the Japanese fungus as causative agent of the panzootic in North America (Hajek et al. Reference Hajek, Humber, Elkinton, May, Walsh and Silver1990) and subsequent elucidation of the E. aulicae species complex (Hajek et al. Reference Hajek, Humber, Walsh and Silver1991) and its narrow host range (Reference Hajek, Humber, Elkinton, May, Walsh and SilverHajek et al. Reference Hajek, Butler, Walsh, Silver, Hain and Hastings1996). Although E. maimaiga was introduced as a biological control agent in various regions of the United States of America (e.g., Smitley et al. Reference Smitley, Bauer, Hajek, Sapiro and Humber1995), no such introductions were done in Canada as the fungus proved quite adapt at naturally colonising and limiting gypsy moth populations along the leading edge of the pest’s distribution (Nealis et al. Reference Nealis, Roden and Ortiz1999; Villedieu and van Frankenhuyzen Reference Villedieu and van Frankenhuyzen2004).
Other entomopathogenic fungi of key interest for pest control are the Fungi Imperfecti, now referred to as Hypocreales, in particular Beauveria bassiana (Balsamo) Vuillemin (Ascomycota: Hypocreales) and Metarhizium anisopliae (Metschnikoff) (Ascomycota: Hypocreales). Isolates of those species account for ~70% of the ~170 commercial mycoinsecticides that have been developed worldwide since the early 1980s, mostly for control of hemipteran and coleopteran pests in agriculture (de Faria and Wraight Reference de Faria and Wraight2007). In contrast to large-scale use for control of Dendrolimus Germar (Lepidoptera: Lasiocampidae) in China, which began in the 1950s and culminated in the early 1980s with treatment of up to one million ha of infested forests annually (Lord Reference Lord2005), this group of fungi has received relatively little attention for control of defoliators in Canada. Spruce budworm is susceptible to various Hypocreales, including B. bassiana, M. anisioplae, and Paecilomyces Samson (now Isaria Persoon) species (Perry et al. Reference Perry, Tyrrell and Strongman1995; Hicks Reference Hicks2007). Plans to develop Isaria farinosa (Holmskjold) Fries (Ascomycota: Hypocreales) for inoculative control of spruce budworm (Perry et al. Reference Perry, Tyrrell and Strongman1995) and later efforts to develop B. bassiana as a mycoinsectide for inundative control of forest Lepidoptera (Hicks Reference Hicks2007) were ultimately not pursued. Hypocrealean fungi were also assessed for control of plantation pests, such as white pine weevil, Pissodes strobi (Peck) (Coleoptera: Curculionidae) (Kope et al. Reference Kope, Alfaro and Lavallée2006, Reference Kope, Alfaro and Lavallée2007; Trudel et al. Reference Trudel, Lavallée, Guertin, Côté, Todorova and Alfaro2007). A novel isolate of B. bassiana (Sabbahi et al. Reference Sabbahi, Lavallée, Merzouki and Guertin2009) is being evaluated for management of introduced invasive beetle pests, including pine shoot beetle, Tomicus piniperda (Linnaeus) (Coleoptera: Curculionidae) (Lavallée et al. Reference Lavallée, Guertin and Thurston2010); emerald ash borer, Agrilus planipennis Fairmaire (Coleoptera: Buprestidae) (Johny et al. Reference Johny, Kyei-Poku, Gauthier and van Frankenhuyzen2012a, Reference Johny, Kyei-Poku, Gauthier, van Frankenhuyzen and Krell2012b); and brown spruce longhorn beetle, Tetropium fuscum (Fabricius) (Coleoptera: Cerambycidae) (Sweeney et al. Reference Sweeney, Silk, Hughes, Lavallée, Blais and Guertin2013). Rather than developing this fungus as a mycoinsecticide, the approach that is currently being evaluated for control of invasive wood-boring pests is augmentation of naturally occurring isolates in the pest’s habitat through deployment of attractant traps containing fungal inoculum to effect dispersal and transmission by the target pest itself (augmentative release) (Lyons et al. Reference Lyons, Lavallée, Kyei-Poku, van Frankenhuyzen, Johny and Guertin2012).
Microsporidia
The name Microsporidia was first proposed by Balbiani in 1882 for spore-forming intracellular parasites found in silk worm (Steinhaus Reference Steinhaus1975). Because Microsporidia were originally considered Protozoa, only recently having been reclassified as belonging to the Kingdom Fungi, much of the work was conducted by parasitologists and protozoologists. Besides early work on species infecting silk worms and honey bees, research on Microsporidia in insects and interest in their use for biological control did not emerge until the 1950s. It was 1961 before the first monograph on insect Microsporidia was published (Weiser Reference Weiser1961, Reference Weiser2005).
Canadian research contributed to accumulation of foundational knowledge through the discovery and description of Microsporidia from a variety of forest insects (Thomson Reference Thomson1959a, Reference Thomson1959b; Smirnoff Reference Smirnoff1966, Reference Smirnoff1975; Wilson and Burke Reference Wilson and Burke1971; Percy et al. Reference Percy, Wilson and Burke1982). Spruce budworm was a key focus, and studies on the life cycle and taxonomy of its most ubiquitous pathogen, Nosema fumiferanae Thomson (Microsporidia: Nosematidae) (Thomson Reference Thomson1955), and how it affects its host (Thomson Reference Thomson1958a) and its transmission and epidemiology (Thomson Reference Thomson1958b) still stand today as the primary source of knowledge on this host-pathogen system. The work was brought to a halt by H.M. Thomson’s untimely death.
Research on Microsporidia was resumed in the early-1970s with the specific goal to explore their potential for biological control through inoculative or inundative release (Wilson et al. Reference Wilson, Tyrrell and Ennis1984). Research addressed dose-mortality relationships (Wilson Reference Wilson1983), in vivo and in vitro production of spores (Sohi and Wilson Reference Sohi and Wilson1976; Wilson Reference Wilson1976), interactions between co-infecting Microsporidia (Wilson Reference Wilson1978), transmission within and between generations (Wilson Reference Wilson1982a) and effects of infection on host fitness (Wilson Reference Wilson1982b; Sanders and Wilson Reference Sanders and Wilson1990). Field trials were conducted in the mid-1970s to evaluate inundative release for spruce budworm control. Mist blower applications of laboratory-produced spores resulted in increased levels of infection, which persisted for about three years in the case of N. fumiferanae and did not carry over to the next year in the case of Pleistophora schubergi Zwölfer (Microsporidia: Nosematidae) (Wilson et al. Reference Wilson, Tyrrell and Ennis1984), a species that differs from the former by not being vertically transmitted (Wilson Reference Wilson1982a, Reference Wilson1982b). The interest in Microsporidia for biological control waned with the lack of field successes and the work was not continued after G.G. Wilson’s departure in the early-1990s.
Limited research on N. fumiferanae did continue with a general focus on its role in spruce budworm population ecology. Earlier studies documented its ubiquitous presence and high prevalence in peak and declining outbreaks (Thomson Reference Thomson1960; Neilson Reference Neilson1963; Wilson Reference Wilson1977). Analysis of spruce budworm population cycles in New Brunswick, Canada lead Royama (Reference Royama1984) to suggest that Nosema Nägeli could play a role in causing population oscillations as part of an unidentified complex of mortality agents acting in concert with parasitism as the primary driver. Later analyses of population trends have not supported such a role (Régnière and Nealis Reference Régnière and Nealis2007, Reference Régnière and Nealis2008). Current knowledge indicates that Nosema-induced direct mortality in outbreak populations is limited (Eveleigh et al. Reference Eveleigh, Lucarotti, McCarthy and Morin2012) and that it likely acts in concert with other ecological factors that affect budworm survival and recruitment, such as foliage depletion (Nealis and Régnière Reference Nealis and Régnière2004), flower production (van Frankenhuyzen et al. Reference van Frankenhuyzen, Ryall, Liu, Meating, Bolan and Scarr2011), spring dispersal of early instars (van Frankenhuyzen et al. Reference van Frankenhuyzen, Nystrom and Liu2007a; Régnière and Nealis Reference Régnière and Nealis2008) and moth dispersal (Eveleigh et al. Reference Eveleigh, Lucarotti, McCarthy, Morin, Royama and Thomas2007).
More than 50 years after its first description, the taxonomy of N. fumiferanae was revisited by using modern molecular tools. Sequencing of ribosomal DNA and various house-keeping genes revealed phylogenetic relationships of Nosema species infecting Choristoneura species and other forest defoliators (Kyei-Poku et al. Reference Kyei-Poku, Gauthier and van Frankenhuyzen2008, Reference Kyei-Poku, Gauthier and van Frankenhuyzen2011b). The combined use of molecular tools and conventional ultra-structure studies is now refining and aiding the discovery and identification of Microsporidia infecting various forest insects (van Frankenhuyzen et al. Reference van Frankenhuyzen, Ebling, McCron, Ladd, Gauthier and Vossbrinck2004; Kyei-Poku et al. Reference Kyei-Poku, Gauthier, Schwarz and van Frankenhuyzen2011a).
Viruses
In the beginning…
Scientists at the Laboratory for Insect Pathology and related Canadian Forest Service laboratories launched the first Canadian research on viral insect diseases in the early-1950s. Susceptibility of insects to infection by viruses was demonstrated in the second decade of the 20th century, amongst others by studies on the wilt disease or “flacheria” of gypsy moth (Glaser and Chapman Reference Glaser and Chapman1912). Although the discovery of polyhedral occlusion bodies in infected insects was made in the 1920s (Steinhaus Reference Steinhaus1975), it was not until the electron microscope became available that virions inside the polyhedra were identified as the infectious agent. Instrumental in that discovery was the work by Bergold (Reference Bergold1947, Reference Bergold1948) in Germany, who was the first to report the presence of virions in occluded viruses from silkworm, gypsy moth, and nun moth, Lymantria monacha (Linnaeus) (Lepidoptera: Erebidae) and by Steinhaus (Reference Steinhaus1948) in the United States of America, who made similar observations on a virus from the alfalfa caterpillar, Colias eurytheme Boisduval (Lepidoptera: Pieridae). Steinhaus tried to recruit Bergold after the Second World War, but his efforts “were thwarted by the U.S. government red tape”, whereas “the Canadians, more adroit in being able to hire aliens – particularly biologists – succeeded” (Steinhaus Reference Steinhaus1975). It was de Gryse who convinced Bergold to join the Canadian Forestry Service in a comprehensive research programme aimed at exploring the potential of insect viruses for control of forest pest insects. This was achieved over the decades that followed through the simultaneous combination of foundational research to identify and characterise viral diseases and applied research to evaluate their effectiveness in forest pest management.
From discovery to genomics
Availability of the electron microscope facilitated discovery and characterisation of occluded viruses from a variety of forest insect species (Table 1). Characterisation initially focussed on virus ultrastructure and biochemistry (Bergold Reference Bergold1953, Reference Bergold1963) and cellular biology of host response and infection processes (Bird Reference Bird1952, Reference Bird1957, Reference Bird1958). Early forays into the biochemistry of viral nucleic acids (Wyatt Reference Wyatt1952) led to a citation by Watson and Crick in their landmark paper on the double helix structure of DNA. Over the decades that followed, biochemical characterisation shifted from physicochemical properties of polyhedra and associated viral particles (Arif and Brown Reference Arif and Brown1975; Bergold Reference Bergold1963) to properties of the DNA itself. Initially genetic characterisation included determination of melting curves, molecular weight, buoyant densities, GC content, and restriction endonuclease profiles (Arif Reference Arif1976; Rohrman et al. Reference Rohrmann, Melgaard, Beaudreau and Martignoni1982; Arif et al. Reference Arif, Guangyu and Jamieson1986; Keddie and Erlandson Reference Keddie and Erlandson1995), the latter permitting differentiation of isolates from various hosts and of closely related genomic variants (Rohrman et al. Reference Rohrmann, McParland, Martignoni and Beaudreau1978; Arif et al. Reference Arif, Guangyu and Jamieson1986; Williams and Otvos Reference Williams and Otvos2005; Zhang et al. Reference Zhang, Lapointe, Thumbi, Morin and Lucarotti2010). The availability of cloning techniques in the 1980s lead to the construction of physical DNA maps for a number of forest insect nucleopolyhedroviruses (Arif et al. Reference Arif, Kuzio, Faulkner and Doerfler1984, Reference Arif, Tjia and Doerfler1985; Arif Reference Arif1986), and characterisation of genes and genomic regions coding for specific polypeptides (Arella et al. Reference Arella, Lavallée, Belloncik and Furuichi1988; Barrett et al. Reference Barrett, Krell and Arif1995; Bah et al. Reference Bah, Bergeron, Arella, Lucarotti and Guertin1997; Echeverry et al. Reference Echeverry, Bergeron, Kaupp, Guertin and Arella1997; Li et al. Reference Li, Barrett, Yuen and Arif1997a, Reference Li, Pang, Lauzon, Sohi and Arif1997b). Critical to these achievements was the parallel development of methods for establishing and culturing permissive insect cell lines (Wyatt Reference Wyatt1956; Sohi and Cunningham Reference Sohi and Cunningham1972; Sohi et al. Reference Sohi, Percy, Arif and Cunningham1984; Sohi Reference Sohi1995; Whittome-Waygood et al. Reference Whittome-Waygood, Fraser, Lucarotti, Otvos, Conder and Levin2009) as systems for virus purification and gene expression (Arif and Pavlik Reference Arif and Pavlik2013).
Table 1 Canadian contributions to the discovery, description, and characterisation of viruses from forest defoliators.

Note: NPV, nucleopolyhedrovirus, Baculoviridae; GV, granulovirus, Baculoviridae; CPV, cypovirus, Reoviridae; EPV, entomopoxvirus, Poxviridae.
The advent of ever more efficient and less expensive sequencing technologies early in the new century facilitated in-depth elucidation of phylogenetic relationships based on gene sequence homologies (Jakubowska et al. Reference Jakubowska, van Oers, Otvos and Vlak2007; Graham et al. Reference Graham, Morin, Lapointe, Nealis and Lucarotti2008; Zhang et al. Reference Zhang, Lapointe, Thumbi, Morin and Lucarotti2010), and eventually sequencing of entire genomes. Approximately 60 baculovirus genomes have been sequenced worldwide with new genome sequences being added at an increasing rate. Complete sequences are known for a variety of forest insect viruses, thanks to collaboration between researchers from government laboratories and universities across Canada, including nucleopolyhedroviruses from C. fumiferana (de Jong et al. Reference de Jong, Lauzon, Dominy, Poloumienko, Carstens and Arif2005; Lauzon et al. Reference Lauzon, Jamieson, Krell and Arif2005), C. freemani Razowski (Lepidoptera: Tortricidae) (Thumbi et al. Reference Thumbi, Beliveau, Cusson, Lapointe and Lucarotti2013), C. rosaceana (Harris) (Lepidoptera: Tortricidae) (Thumbi et al. Reference Thumbi, Beliveau, Cusson, Lapointe and Lucarotti2013), Orgyia leucostigma (Smith) (Lepidoptera: Erebidae) (Thumbi et al. Reference Thumbi, Eveleigh, Lucarotti, Lapointe, Graham and Pavlik2011), Neodiprion sertifer (Geoffroy) (Hymenoptera: Diprionidae) (Garcia-Maruniak et al. Reference Garcia-Maruniak, Maruniak, Zanotto, Doumbouya, Liu and Merritt2004; Lauzon et al. Reference Lauzon, Garcia-Maruniak, de Azanotto, Clemente, Herniiou and Lucarotti2006), N. lecontei (Fitch) (Hymenoptera: Diprionidae) (Lauzon et al. Reference Lauzon, Lucarotti, Krell, Feng, Retnakaran and Arif2004, Reference Lauzon, Garcia-Maruniak, de Azanotto, Clemente, Herniiou and Lucarotti2006), and N. abietis (Harris) (Hymenoptera: Diprionidae) (Duffy et al. Reference Duffy, Young, Morin, Lucarotti, Koop and Levin2006); granulovirus from C. fumiferana and C. freemani (Escasa et al. Reference Escasa, Lauzon, Mathur, Krell and Arif2006); a cypovirus from C. freemani (Graham et al. Reference Graham, Morin, Lapointe, Nealis and Lucarotti2008); and entomopoxviruses from C. biennis Freeman and C. rosaceana (Thézé et al. Reference Thézé, Takatsuka, Li, Gallais, Doucet and Arif2013). Genomic sequence homologies are now being applied to elucidate the molecular basis of target insect specificity (Lauzon et al. Reference Lauzon, Garcia-Maruniak, de Azanotto, Clemente, Herniiou and Lucarotti2006) and to facilitate regulatory approval of viruses as forest insect control products (Lapointe et al. Reference Lapointe, Thumbi and Lucarotti2012).
Development of virus-based control products
Much of the success of baculoviruses for control of forest insects in Canada depended on a concomitant and concerted field evaluation of their potential as control agents. The first field trials took place in the early-1950s in southern Ontario with a nucleopolyhedrovirus from the European pine sawfly, N. sertifer, which was brought in from Sweden and propagated in the laboratory (Bird Reference Bird1953). In the decades that followed, numerous field tests were undertaken to examine baculoviruses for control of eruptive forest insects in more than 20 different baculovirus/host systems (as reviewed by Smirnoff and Juneau Reference Smirnoff and Juneau1973; Cunningham and Kaupp Reference Cunningham and Kaupp1995; Wallace and Cunningham Reference Wallace and Cunningham1995; Cunningham Reference Cunningham1998). In those investigations, baculovirus occlusion bodies were typically obtained by infection of laboratory-reared larvae (in the case of lepidopteran defoliators) or by harvesting dead larvae after treatment of heavily infested stands (in the case of hymenopteran defoliators), followed by grinding of freeze-dried cadavers to a fine powder. The occlusion bodies were suspended in a water solution containing synthetic stickers and other additives, and applied with mist blowers, backpack sprayers, or aircraft, using pest-specific and product-specific application prescriptions, as reviewed by Cunningham and Entwistle (Reference Cunningham and Entwistle1981) and van Frankenhuyzen et al. (Reference van Frankenhuyzen, Reardon and Dubois2007b). Baculovirus introductions proved to be very effective for suppression of eruptive forest insect populations in many of those tests (Cunningham Reference Cunningham1998).
Field evaluation of baculoviruses for population control was underpinned by years of basic research on their prevalence (Laitinen et al. Reference Laitinen, Otvos and Levin1996; Lucarotti et al. Reference Lucarotti, Eveleigh, Royama, Morin, McCarthy and Ebling2004; van Frankenhuyzen et al. Reference van Frankenhuyzen, Ebling, Thurston, Lucarotti, Royama and Guscott2005), transmission (Bird Reference Bird1961; Smirnoff Reference Smirnoff1962b; Roland and Kaupp Reference Roland and Kaupp1995; Cory and Myers Reference Cory and Myers2003; Graves et al. Reference Graves, Lucarotti and Quiring2012a, Reference Graves, Quiring and Lucarotti2012b), and role (Neilson Reference Neilson1963; Myers Reference Myers2000; Cooper et al. Reference Cooper, Cory, Theilmann and Myers2003) in forest insect outbreaks and concomitant laboratory characterisation of their virulence (Stairs Reference Stairs1965; Ebling and Kaupp Reference Ebling and Kaupp1997; Ebling et al. Reference Ebling, Barrett and Arif1998; Ebling et al. Reference Ebling, Otvos and Conder2004). The role of baculoviruses in host population dynamics varies from species to species. In the case of spruce budworm, baculoviruses typically remain at low prevalence during an outbreak cycle (Lucarotti et al. Reference Lucarotti, Eveleigh, Royama, Morin, McCarthy and Ebling2004) as naturally occurring epizootics have never been reported (Cunningham and Kaupp Reference Cunningham and Kaupp1995). In contrast, viral epizootics are often associated with population declines in tussock moths (Otvos et al. Reference Otvos, Cunningham and Shepherd1995; van Frankenhuyzen et al. Reference van Frankenhuyzen, Ebling, Thurston, Lucarotti, Royama and Guscott2005), tent caterpillars (Myers Reference Myers2000), gypsy moths (Reference Hajek, Humber, Elkinton, May, Walsh and SilverHajek Reference Hajek1997), and sawflies (Bird and Elgee Reference Bird and Elgee1957; Smirnoff Reference Smirnoff1972; Oloffson Reference Oloffson1987; Wallace and Cunningham Reference Wallace and Cunningham1995). It is therefore not surprising that the use of baculoviruses for management of outbreak populations has been most successful for species with population cycles that are primarily driven by indigenous viral disease, specifically sawflies (Wallace and Cunningham Reference Wallace and Cunningham1995; Moreau et al. Reference Moreau, Lucarotti, Kettela, Thurston, Holmes and Weaver2005), Douglas-fir tussock moth (Orgyia pseudotsugata (McDunnough); Lepidoptera: Erebidae) (Otvos and Shepherd Reference Otvos and Shepherd1991; Otvos et al. Reference Otvos, Cunningham and Shepherd1995), whitemarked tussock moth, (O. leucostigma) (Embree et al. Reference Embree, Elgie and Estabrooks1984), and gypsy moth (Cunningham et al. Reference Cunningham, Kaupp and Howse1991, Reference Cunningham, Kaupp, Fleming, Brown and Burns1993). Augmentation of indigenous viral disease through spray application can not only control (Cunningham et al. Reference Cunningham, Kaupp and Howse1991, Reference Cunningham, Kaupp, Fleming, Brown and Burns1993) but also terminate (Moreau et al. Reference Moreau, Lucarotti, Kettela, Thurston, Holmes and Weaver2005) and even prevent (Otvos et al. Reference Otvos, Cunningham and Alfaro1987) outbreaks of those species.
Field successes lead to the development and registration of several baculovirus products by the Canadian Forest Service between the early-1980s and early-2000s (Table 2). Before the start of the new millennium, products were produced in-house, using methods that were tailored to optimise yield and virulence (Kaupp and Ebling Reference Kaupp and Ebling1993; Ebling and Kaupp Reference Ebling and Kaupp1998, Reference Ebling and Kaupp1999; Otvos et al. Reference Otvos, Kukan, Reardon and Ragenovich2006; Thorne et al. Reference Thorne, Levin, Otvos and Conder2008). However, baculovirus products were not used for operational pest control because there were no commercial products. Efforts to facilitate baculovirus commercialisation focussed initially on the nucleopolyhedrovirus of gypsy moth, which was first registered as GypchekTM in the United States of America and later as DisparvirusTM in Canada (Cunningham Reference Cunningham1998), and which became the target for a commercial pilot production facility in Sault Ste. Marie funded by American Cyanamid during the early-1990s. When the company pulled out, the Canadian Forest Service attempted to promote commercialisation by transferring product registrations to private industries, but to no avail. High production costs, resulting from the need for in vivo production, and market limitations resulting from high target specificity combined with the cyclical nature of target pest outbreaks and multi-year treatment efficacy, made baculovirus forestry products unattractive for private investment.
Table 2 Baculoviruses registered in Canada for use against forest insect pests.

Commercialisation
A different path was followed for the development and commercialisation of AbietivTM, a product containing the nucleopolyhedrovirus of the balsam fir sawfly, N. abietis. A sustained outbreak of this pest in Newfoundland during the 1990s precipitated a multi-year partnership between governments, private industry, and academia to investigate the pest’s ecology and impact and to explore options for biological control (Lucarotti et al. Reference Lucarotti, Moreau and Kettela2007a, Reference Lucarotti, Morin., Graham and Lapointe2007b). A nucleopolyhedrovirus was isolated from a local population and mass-produced in the field for further characterisation (Duffy et al. Reference Duffy, Young, Morin, Lucarotti, Koop and Levin2006, Reference Duffy, Becker, Whittome, Lucarotti and Levin2007; Graves et al. Reference Graves, Quiring and Lucarotti2012b; Lucarotti et al. Reference Lucarotti, Whittome-Waygood, Lapointe, Morin and Levin2012), field evaluation on ~22 000 ha between 2001 and 2005 (Moreau et al. Reference Moreau, Lucarotti, Kettela, Thurston, Holmes and Weaver2005; Moreau and Lucarotti Reference Moreau and Lucarotti2007) and environmental safety testing. The Canadian Forest Service obtained conditional registration in 2006 and full registration in 2009 of AbietivTM for control of balsam fir sawfly. A licensing agreement with a newly formed company, Sylvar Technologies Inc. (Fredericton, New Brunswick, Canada), led to the first operational use of a commercially produced baculovirus product in our history of forest pest management. AbietivTM was used for control of balsam fir sawfly in Newfoundland on 15 000 ha each in 2006 and 2007, 10 000 ha in 2008, and 5000 ha in 2009, and on 10 000 ha in New Brunswick in 2011. The licensing agreement with Sylvar Technologies was subsequently expanded to include DisparvirusTM, VirtussTM, and LecontvirusTM (Table 2). In 2011, a 60% controlling interest in Sylvar Technologies was acquired by an international biocontrol company, Andermatt Biocontrol AG (Grosdietwil, Switzerland), which added the forestry products to a suite of baculovirus products targeted against agricultural pests. So after more than 60 years of government investment in research and development, baculovirus products for forest insect control are now in the hands of commercial interests, a development that is hoped to sustain and expand their production and availability for operational use into the future.
Despite inconsistent availability, baculovirus products have been applied to tens of thousands of hectares of insect-infested forests since the onset of field testing in the 1950s. Such use has to date met with broad general acceptance by the Canadian public due to several key features. Viruses that are used in forest pest control products are ubiquitous in terrestrial and aquatic environments and occur naturally in the target pest populations. They lack infectivity to organisms outside their extremely narrow host range, which is limited to one (GypchekTM, DisparvirusTM, LecontvirusTM, AbietivTM) or a few closely related species in the same genus (VirtussTM). There have been no reports of negative impacts of their use in forest protection on ecosystems other than the effect on the target host species, as reviewed by Lapointe et al. (Reference Lapointe, Thumbi and Lucarotti2012). Because of their natural role in pest population cycles, their high degree of target specificity, and effects across pest generations, baculoviruses are generally considered to be the ecologically most responsible forest pest control option available to date.
Bacteria
Among the scientists recruited to staff the Laboratory for Insect Pathology were several bacteriologists. Their search for bacteria that could be conscripted in the war against the spruce budworm quickly focussed on Bacillus thuringiensis Berliner (Firmicutes: Bacillaceae), a bacterium that had been described in 1915 as a pathogen of flour moth, and which had been used against corn borer in Europe during the late-1920s and early-1930s. The first commercial product in North America (ThuricideTM) was produced by the Bioferm Corporation in California, United States of America and became available in 1957 (Steinhaus Reference Steinhaus1975), around the same time that Canadian scientists started to consider the use of this pathogen for spruce budworm control. Canadian research over the 35 years that followed shaped B. thuringiensis into a commercially viable and effective alternative to conventional insecticides for control of many lepidopteran forest pests. Success of B. thuringiensis in the Canadian forestry market became the basis for much broader subsequent development worldwide as a biopesticide in forestry, agriculture, and human health (van Frankenhuyzen Reference van Frankenhuyzen1993).
Early mode of action research
Research in the 1950s and 1960s focussed on mode of action of B. thuringiensis. Pivotal contributions include the discoveries that: (i) parasporal bodies formed during sporulation are protein crystals (Hannay Reference Hannay1953); (ii) crystals are responsible for larval toxicity (Angus Reference Angus1954); (iii) solubilisation of crystals under alkaline conditions releases toxin proteins which change ion permeability of midgut cell membranes (Heimpel and Angus Reference Heimpel and Angus1959; Fast and Angus Reference Fast and Angus1965; Fast and Morrison Reference Fast and Morrison1972); (iv) crystal proteins bind to the cell surface (Murphy et al. Reference Murphy, Sohi and Fast1976); and (v) spores and spore-associated virulence factors enhance the toxic effects of crystal protein by causing septicemia and accelerating larval death (Heimpel and Angus Reference Heimpel and Angus1959; Fast Reference Fast1977). Other work explored diversity of B. thuringiensis isolates and their pathogenicity (Heimpel and Angus Reference Heimpel and Angus1960; Smirnoff Reference Smirnoff1965; Yamvrias and Angus Reference Yamvrias and Angus1970), biochemistry and serology of delta-endotoxins (Krywienczyk and Angus Reference Krywienczyk and Angus1967; Fast and Angus Reference Fast and Angus1970; Krywienczyk et al. Reference Krywienczyk, Dulmage, Hall, Beegle, Arakawa and Fast1981; Fast Reference Fast1983), and histopathology of intoxication (Heimpel and Angus Reference Heimpel and Angus1959; Percy and Fast Reference Percy and Fast1983). This work laid the foundation for a knowledge base that expanded rapidly in ensuing years as the pest control potential of B. thuringiensis started to capture the interest of research laboratories and pesticide manufacturers around the world.
From first field tests to operational acceptance
At the same time that foundational research was being conducted, Canadian Forest Service scientists spearheaded a concerted field development effort starting in the early-1960s. Mounting concerns about the environmental impact of yearly spray operations with dichlorodiphenyltrichloroethane in eastern Canada resulted in political pressure to field test B. thuringiensis as a possible alternative, a step that was at that time considered by the principal investigator (T.A. Angus) to be highly premature. The availability of ThuricideTM ushered in a decade of intermittent field testing, with generally inconsistent and inadequate results (Morris et al. Reference Morris, Angus and Smirnoff1975). Two developments were of particular significance in improving efficacy and accelerating commercialisation: the discovery and adoption of the superior kurstaki isolate of HD-1 for commercial production in the early-1970s, and the concomitant adoption of the International Unit for standardisation of product quality and potency (van Frankenhuyzen Reference van Frankenhuyzen1995). Commercial products improved during the 1970s and facilitated extensive field testing under auspices of the CANUSA programme, a collaboration of industry and agencies at various levels of government on both sides of the border. New formulations were tested and application prescriptions were developed in terms of hardware (types of aircraft and spray dispersal systems), timing, and frequency of treatment, and application rates required to increase spray deposition (Smirnoff Reference Smirnoff1980) and efficacy (Smirnoff and Morris Reference Smirnoff and Morris1982; Morris Reference Morris1984). Although effectiveness of B. thuringiensis sprays improved markedly over time, results remained inconsistent and treatment costs were much higher than with chemical insecticides (van Frankenhuyzen Reference van Frankenhuyzen1995).
Operational use in the early-1980s before cost and efficacy were competitive with chemical insecticides catalysed significant cost reductions and provided the experience to improve efficacy. Cost effectiveness started to improve as formulations became more concentrated and suitable for undiluted application in volumes as low as 2.4 L per ha. This not only increased spray aircraft efficiencies but also reliability of treatment efficacy (Lewis et al. Reference Lewis, Walton, Dimond, Morris, Parker and Reardon1984; Morris et al. Reference Morris, Dimond and Lewis1984; Dorais Reference Dorais1985; Kettela Reference Kettela1985; Smirnoff Reference Smirnoff1985), while large-scale use and competitive bidding forced the product cost further down. By the mid-1980s, product cost, application cost, and efficacy were not far from being at par with the use of the organophosphate FenitrothionTM, which by then had become the mainstay of forest protection in Canada. Cost reductions opened the door for provincial jurisdictions to favour B. thuringiensis over synthetic insecticides in response to mounting public opposition to chemical sprays. As a result, between 1985 and 1990 B. thuringiensis products gradually replaced synthetic insecticides in spruce budworm control programmes across Canada (van Frankenhuyzen Reference van Frankenhuyzen2000).
In most of Canada, B. thuringiensis is now the only agent used for management of lepidopteran forest defoliators. Between 1985 and 2012, commercial products were applied on a cumulative total of about 10 million ha of insect-infested forests, primarily to prevent excessive defoliation by spruce budworm, other budworm species, gypsy moth, and eastern hemlock looper (Fig. 2). Its use declined sharply in the early-1990s as the spruce budworm outbreak in eastern Canada collapsed, and shifted towards western provinces for control of both eastern and western spruce budworms (Fig. 2). The steep increase in area sprayed between 2007 and 2012 reflects the recurrence of epidemic spruce budworm populations in Québec, which is viewed as the onset of a new outbreak that is expected to once again sweep across eastern North America.
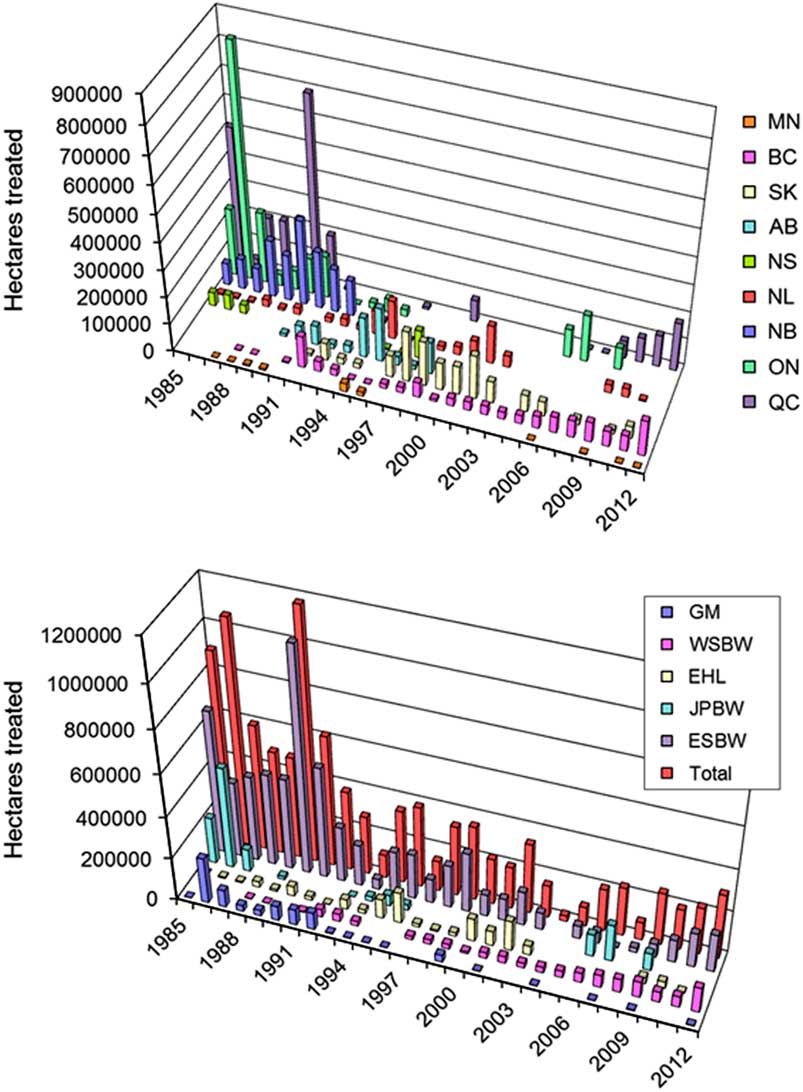
Fig. 2 Operational use (total hectares treated) of B. thuringiensis for control of defoliating forest Lepidoptera across Canada between 1985 and 2012. Data for these figures were obtained from provincial forest protection agencies and compiled by the lead author. Top panel: Use by province (MN, Manitoba; BC, British Columbia; SK, Saskatchewan; AB, Alberta; NS, Nova Scotia; NL, Newfoundland and Labrador; NB, New Brunswick; QC, Québec; ON, Ontario)Bottom panel: Use by major pest (GM, gypsy moth, Lymantria dispar; WSBW, western spruce budworm, Choristoneura freemani; EHL, eastern hemlock looper, Lambdina fiscellaria fiscellaria; JPBW, jack pine budworm, Choristoneura pinus; ESBW, eastern spruce budworm, Choristoneura fumiferana)
Large-scale operational use of B. thuringiensis in forestry has been characterised by broad public acceptance and preference over the use of synthetic insecticides (Chang et al. Reference Chang, Lantz and Maclean2009). The adoption of B. thuringiensis in the context of public opposition to large-scale aerial application of chemical insecticides no doubt contributes to this acceptance. Although not as target-specific as baculoviruses, B. thuringiensis products have high specificity compared to broader spectrum organophosphates. Public acceptance is further facilitated by numerous studies showing that effects on non-target organisms and indirect effects on forest ecosystem processes are either nonexistent or limited and temporary (e.g., Kreutzweiser et al. Reference Kreutzweiser, Holmes, Capell and Eichenberg1992, Reference Kreutzweiser, Gringorten, Thomas and Butcher1996; Addison Reference Addison1993; Addison and Holmes Reference Addison and Holmes1995, Reference Addison and Holmes1996; Holmes Reference Holmes1998; Addison et al. Reference Addison, Otvos, Battigelli and Conder2006). Large-scale use in urban settings, for example for control of introduced invasive pests, is a more contentious issue (Ginsburg Reference Ginsburg2006) despite studies showing no public health impacts of aerial applications in Canada (Pearce et al. Reference Pearce, Habbick, Williams, Eastman and Newman2002) or elsewhere (Green et al. Reference Green, Heumann, Solokow, Foster, Bryant and Skeels1990).
Reducing efficacy constraints
Operational use of B. thuringiensis for management of defoliating forest insects went hand in glove with research to increase both efficacy and efficiency of spray programmes. Much of that research was conducted under auspices of an association of agencies and industries that have a stake in forest pest management, which is now called the Spray Efficacy Research Group-International (SERG-I). SERG-I started in the early 1980s as a New Brunswick-based cooperative aimed at improving efficacy of aerial pesticide spraying. Lessons learned from aerial application of chemical insecticides, in particular the importance of controlled droplet application and use of ultra-low spray volumes, were readily transferred to B. thuringiensis once it became operationally accepted (van Frankenhuyzen Reference van Frankenhuyzen1995). That required modified timing of application, considering that the agent has to be ingested to be effective and that it has limited residual toxicity (van Frankenhuyzen and Nystrom Reference van Frankenhuyzen and Nystrom1989). It also required a trade-off between minimum emitted droplet size and product potency (van Frankenhuyzen and Payne Reference van Frankenhuyzen and Payne1993) to ensure delivery of a lethal dose in operationally attainable spray deposits of one or two droplets per needle (Payne and van Frankenhuyzen Reference Payne and van Frankenhuyzen1995) and to minimise temporary inhibition of feeding caused by ingestion of sublethal doses (Fast and Régnière Reference Fast and Régnière1984; van Frankenhuyzen Reference van Frankenhuyzen1990). Those interactions were eventually integrated into a process-oriented, model to simulate efficacy of B. thuringiensis sprays against spruce budworm (Cooke and Régnière Reference Cooke and Régnière1996). Field validation of the model (Régnière and Cooke Reference Régnière and Cooke1998) supports the notion that current understanding of interacting processes that underlie the efficacy of B. thuringiensis against spruce budworm is reasonably complete. Subsequent testing of model predictions in multi-year experimental programmes in the late-1990s in Québec under a range of operational conditions led to current application and treatment prescriptions for optimisation of foliage protection (Bauce et al. Reference Bauce, Carisey, Dupont and van Frankenhuyzen2004).
Reducing efficiency constraints
Increasing aerial spray programme efficiency was the second main thrust behind 30 years of research supported by SERG-I, an effort that was driven by Forest Protection Limited in New Brunswick and other forest protection agencies across eastern Canada, with participation of companies, universities, federal and provincial governments, and the United States Department of Agriculture, Forest Service. Although key accomplishments of this research pertain to forestry application of pesticides in general, they have been and are being applied primarily to the use of B. thuringiensis, and are therefore briefly reviewed below.
Major contributions to refining efficiency of foliage protection programmes came from advancements in aerial application technology and electronic guidance systems that were attained from extensive research conducted during spruce budworm control programmes in the 1980s and early-1990s. Advancements culminated in the AccuairTM Aerial Management (AAM) System (McLeod et al. Reference McLeod, Lucarotti, Hennigar, MacLean, Holloway, Cormier and Davies2012), a sophisticated onboard guidance and control system that optimises flight lines on a spray block to compensate for changes in wind direction and aircraft altitude while spraying. The AAM system is the result of 30 years of modelling and field validation on how to minimise off-target drift and maximise on-target deposition of droplets in the size range used in ultra-low-volume forestry applications (Picot et al. Reference Picot, Bontemps and Kristmanson1985, Reference Picot, Kristmanson and Basak-Brown1986; van Vliet and Picot Reference van Vliet and Picot1987; Crabbe and McGooeye Reference Crabbe and McCooeye1995; Wiesner Reference Wiesner1995). By linking real-time recordings of spray aircraft position and near-canopy meteorological conditions with a spray droplet dispersion model to predict down-wind spray deposition, the system makes real-time predictions for spray aircraft flight paths that will maximise on-target spray coverage. Field validation has shown that AMM allows accurate application of B. thuringiensis and other pesticides to small blocks of complex shape. It is now used in combination with an auto-flow system that automatically adjusts pesticide flow rate through the atomisers for changes in aircraft ground speed in order to reduce variability in application rate (McLeod et al. Reference McLeod, Lucarotti, Hennigar, MacLean, Holloway, Cormier and Davies2012).
Another key contribution was the development during the 1990s of the spruce budworm decision support system, which permits users to determine the effects of different foliage protection scenarios on marginal timber supply benefits (MacLean et al. Reference MacLean, Erdle, MacKinnon, Porter, Beaton and Cormier2001, Reference MacLean, Beaton, Porter, MacKinnon and Budd2002). Subsequent improvements and integration of other spatial and non-spatial tools produced the AccuairTM Forest Protection Optimization System (ForPRO), a software package that allows users to better target and optimise both direct and indirect economic benefits of forest protection programmes, and to simulate impacts of spruce budworm and other insect outbreaks and planned foliage protection programmes on stand and forest development (Chang et al. Reference Chang, Lantz, Hennigar and MacLean2011; Hennigar et al. Reference Hennigar, MacLean, Porter and Quiring2007; Iqbal et al. Reference Iqbal, Hennigar and MacLean2012). Integration of ForPro with AAM for management of spruce budworm and other forest insects has eliminated the need for the large spray blocks that characterised operational programmes during the 1980s and 1990s. Forest inventory and stand information are now used to target protection programmes to vulnerable stands that are most in need of protection, while advanced navigation and control systems enable efficient targeting of blocks with irregular shape and size that typically result from that process (McLeod et al. Reference McLeod, Lucarotti, Hennigar, MacLean, Holloway, Cormier and Davies2012).
Mode of action research in the molecular era
Changing social attitudes towards pesticide use during the 1980s converged with the advent of recombinant DNA technology, leading to a dramatic increase in the interest in B. thuringiensis as a biopesticide around the world. The demonstration in Canadian forestry that B. thuringiensis as an operational tool could indeed compete with synthetic insecticides in terms of cost and efficacy was pivotal in drawing the world’s attention to the natural diversity of B. thuringiensis strains as a source for socially acceptable pest control products in other markets (van Frankenhuyzen Reference van Frankenhuyzen1993). Cloning of the first crystal protein genes in the early-1980s opened the door for exploiting the diversity of B. thuringiensis pesticidal proteins, and led to the application of powerful molecular tools that revolutionised mode of action research.
Against this background of rapidly developing new technologies, P.G. Fast from the Canadian Forest Service recognised the need for and merits of a multidisciplinary approach for further unravelling B. thuringiensis toxin mode of action. To that end he established in the early-1980s the Biocide research network by engaging scientists from a broad array of disciplines in federal government, universities, and National Research Council laboratories. That engagement lasted well beyond the ~10 years of formal collaboration into the new millennium. By integrating a suite of modern technologies, Biocide participants have made key contributions to understanding toxin mode of action, particularly in the areas of crystal protein chemistry, receptor binding, and pore formation.
Notable early advances in crystal protein chemistry include the use of Raman spectroscopy to shed light on sunlight-inactivation of crystal protein (Pozsgay et al. Reference Pozsgay, Fast, Kaplan and Carey1987; Puzstai et al. Reference Puzstai, Fast, Gringorten, Kaplan, Lessard and Carey1991) and X-ray diffraction to decipher for the first time the three-dimensional structure of a Lepidoptera-active crystal protein (Grochulski et al. Reference Grochulski, Masson, Borisova, Puzstai-Carey, Schwartz and Brousseau1995). Pusztai-Carey et al. (Reference Pusztai-Carey, Carey, Lessard and Yaguchi1995) developed a method to separate, identify, and purify crystal proteins by using high pressure liquid chromatography, which has become the international gold standard for studies on a variety of topics, such as crystal protein structure-function relationships (Grochulski et al. Reference Grochulski, Masson, Borisova, Puzstai-Carey, Schwartz and Brousseau1995; Padilla et al. Reference Padilla, Pardo-Lopez, De La Riva, Gomez, Sanchez and Hernandez2006), specificity (Monnerat et al. Reference Monnerat, Masson, Brousseau, Puzstai-Carey, Bordat and Frutos1999), resistance (Anilkumar et al. Reference Anilkumar, Rodrigo-Simon, Ferré, Puzstai-Carey, Sivasupramaniam and Moar2008), and non-target effects (Hilbeck et al. Reference Hilbeck, Moar, Puzstai-Carey, Filippini and Bigler1999; Kramarz et al. Reference Kramarz, De Vaufleury and Carey2007). Other technologies were applied to garner novel insights in crystal protein binding to receptors on the surface of midgut cells, and in formation of ion channels by postbinding insertion of toxin molecules into the cell membrane (generally referred to as pore formation), processes which are critical in determining toxicity and target specificity. Surface plasmon resonance revealed kinetics of toxin-receptor interactions (Masson et al. Reference Masson, Mazza and Brousseau1994, Reference Masson, Lu, Mazza, Brousseau and Adang1995a, Reference Masson, Mazza, Sangadala, Adang and Brousseau2002a) and proved useful in unraveling mechanisms of resistance to crystal proteins (Masson et al. Reference Masson, Mazza, Brousseau and Tabashnik1995b; Luo et al. Reference Luo, Sangadala, Masson, Mazza, Brousseau and Adang1997; Tabashnik et al. Reference Tabashnik, Liu, Malvar, Heckel, Masson and Ferré1998), while atomic force microscopy and Fourier transform infra-red spectroscopy were used to visualise for the first time the insertion of toxin molecules into cell membranes (Vie et al. Reference Vie, van Mau, Pomarède, Dance, Schwartz and Laprade2001; Laflamme et al. Reference Laflamme, Badia, Lafleur, Schwartz and Laprade2008).
Further insights into the complex process of pore formation were obtained by combining electrophysiology with cell physiology and molecular genetics. Pore formation was studied with micro-electrophysiological techniques measuring toxin-induced changes in membrane potential of either epithelial cells in whole larval midgut preparations (“patch-clamping”; Peyronnet et al. Reference Peyronnet, Vachon, Brousseau, Baines, Schwartz and Laprade1997) or of artificial lipid bilayers (“planar lipid bilayers”; Schwartz et al. Reference Schwartz, Garneau, Savaria, Masson, Brousseau and Rousseau1993, Reference Schwartz, Lu, Söhnlein, Brousseau, Laprade and Masson1997a). Pore formation was also studied using cultured insect cells (Schwartz et al. Reference Schwartz, Garneau, Masson and Brousseau1991; Potvin et al. Reference Potvin, Laprade and Schwartz1998; Villalon et al. Reference Villalon, Vachon, Brousseau, Schwartz and Laprade1998) and epithelial cell preparations obtained from homogenised insect midguts (brushborder membrane vesicles; Peyronnet et al. Reference Peyronnet, Vachon, Schwartz and Laprade2001). Studies using these techniques revealed for the first time the primary role of calcium and chloride in toxin action (Schwartz et al. Reference Schwartz, Garneau, Masson and Brousseau1991) and the presence of endogenous ion channels in the apical membrane of epithelial cells (Peyronnet et al. Reference Peyronnet, Noulin, Laprade and Schwartz2004), and elucidated the influence of various biophysical and biochemical factors on pore formation (Fortier et al. Reference Fortier, Vachon, Kirouac, Schwartz and Laprade2005, Reference Fortier, Vachon, Frutos, Schwartz and Laprade2007; Vachon et al. Reference Vachon, Schwartz and Laprade2006; Brunet et al. Reference Brunet, Vachon, Marsolais, van Rie, Schwartz and Laprade2010b). In combination with site-directed mutagenesis, those techniques permitted probing the importance of specific toxin regions or amino acid residues in pore formation. Systematic replacement of individual amino acids revealed the involvement of specific domains (Schwartz et al. Reference Schwartz, Potvin, Chen, Brousseau, Laprade and Dean1997b; Masson et al. Reference Masson, Tabashnik, Mazza, Préfontaine, Potvin and Brousseau2002b), interdomain salt bridges (Coux et al. Reference Coux, Vachon, Rang, Moozar, Masson and Royer2001), specific α-helices (Masson et al. Reference Masson, Tabashnik, Yong-Biao, Brousseau and Schwartz1999; Vachon et al. Reference Vachon, Préfontaine, Coux, Rang, Marceau and Masson2002, Reference Vachon, Préfontaine, Rang, Coux, Juteau and Schwartz2004; Girard et al. Reference Girard, Vachon, Préfontaine, Marceau, Schwartz and Masson2009), and interhelical loops ( Lebel et al. Reference Lebel, Vachon, Préfontaine., Girard, Masson and Juteau2009; Brunet et al. Reference Brunet, Vachon, Marsolais, Arnaut, van Rie and Marceau2010a). A recent synthesis of results from this work and from related studies elsewhere in the world has yielded the most parsimonious and empirically best supported model of B. thuringiensis crystal protein mode of action that is available to date (Vachon et al. Reference Vachon, Laprade and Schwartz2012).
Conspicuous in the molecular era of mode of action research is the declining role of Canadian Forest Service scientists. With full operationalisation of B. thuringiensis in forest protection during the preceding decades, the product development phase that was initiated by the Canadian Forest Service 60 years earlier came to an end. As B. thuringiensis attained “mature product” status, the federal government gradually reduced its related research investments. Besides some work on spore-associated virulence factors (Kyei-Poku et al. Reference Kyei-Poku, Gauthier, Pang and van Frankenhuyzen2007; Milne et al. Reference Milne, Liu, Gauthier and van Frankenhuyzen2008; Kalmykova et al. Reference Kalmykova, Burtseva, Milne and van Frankenhuyzen2009), interactions with midgut microbes (van Frankenhuyzen et al. Reference van Frankenhuyzen, Liu and Tonon2010) and recent syntheses of crystal protein specificity (van Frankenhuyzen Reference van Frankenhuyzen2009, Reference van Frankenhuyzen2013), federally funded research on B. thuringiensis has virtually ground to a halt.
Forest pest control with B. thuringiensis in the molecular era
Cloning of the first crystal protein genes not only revolutionised research on the mode of action of B. thuringiensis, but also its application in pest control. The insertion of crystal protein genes in plants to produce insect-resistant transgenic crops has found widespread adoption in agricultural production around the world since its introduction in the mid-1990s. Advances in technologies for in vitro propagation and genetic transformation of various tree species accelerated the development of transgenic forest trees during the 1990s. Using B. thuringiensis crystal protein genes, insect resistance has been engineered into several tree species, including poplar (Populus Linnaeus; Salicaceae), walnut (Juglans Linnaeus; Juglandaceae), larch (Larix Miller; Pinaceae), eucalyptus (Eucalyptus L’Héritier de Brutelle; Myrtaceae), and white spruce (Picea glauca (Moench) Voss; Pinaceae), as reviewed by van Frankenhuyzen and Beardmore (Reference van Frankenhuyzen and Beardmore2004). In Canada, the development of efficient methods for transformation and somatic embryogenesis of spruces permitted the creation of a spruce budworm-resistant white spruce, which was tested in a confined field trial (Lachance et al. Reference Lachance, Hamel, Pelletier, Valéro, Bernier-Cardou and Chapman2007). The key objective of that project was not to design budworm-resistant trees for commercial plantations, but to develop proof of concept in conifer genetic engineering using insect resistance as a model silvicultural trait. Bt-spruce also served as a model system for the development of protocols to evaluate environmental effects of transgene applications (Lamarche and Hamelin Reference Lamarche and Hamelin2007; Leblanc et al. Reference LeBlanc, Hamelin and Filion2007). Canadian scientists are now playing a role in defining an international framework for proper evaluation of commercial release of transgenic trees (Häggman et al. Reference Häggman, Raybould, Borem, Fox, Handley and Hertzberg2013). Transgene applications of B. thuringiensis crystal proteins in forestry may never be desirable, considering the myriad of ecological risks associated with their deployment (van Frankenhuyzen and Beardmore Reference van Frankenhuyzen and Beardmore2004), not the least of which is the induction of resistance in key target pests such as the spruce budworm (van Frankenhuyzen et al. Reference van Frankenhuyzen, Nystrom and Tabashnik1995).
The future of insect pathology in Canada
The preceding synopsis documents key contributions made by Canadian scientists to the study of forest insect diseases from when insect pathology was first established as a discipline in its own right until today, a period of about 65 years. Developments in Canada over that period largely reflected the development of the discipline elsewhere in the world. The emerging promise of insect pathogens for sustainable and ecologically acceptable control of insect pests around the middle of the previous century led to active research programmes in insect diseases in many countries (Steinhaus Reference Steinhaus1975). This effort resulted in the development of pathogen-based approaches for control of a broad range of insect pests around the world, including products based on B. thuringiensis (Charles et al. Reference Charles, Delécluse and Nielsen-LeRoux2000), baculoviruses (Hunter-Fujita et al. Reference Hunter-Fujita, Entwistle, Evans and Crook1998) and Hypocreales fungi (de Faria and Wraight Reference de Faria and Wraight2007), as well as classical biological control using insect pathogens ((Reference Hajek, Humber, Elkinton, May, Walsh and SilverHajek Reference Hajek, Humber, Elkinton, May, Walsh and Silverand Delalibera Reference Hajek and Delalibera2010).
Much of the research in Canada was driven by the desire to develop products that could replace broad-spectrum synthetic insecticides in aerial forestry applications. As a result, investigations focussed primarily on pathogens that could be mass-produced, stored, and formulated for aerial spray application. Bacillus thuringiensis and baculoviruses met those criteria and became prime candidates for development as control products that fit this chemical control paradigm. That phase of insect pathology research may now have come to an end: the “low-hanging fruit” has been picked, and most likely candidates have been developed to commercialisation. There are no doubt other agents that can be developed as pest control products, in particular other baculoviruses, but such activities are now likely to fall within the purview of private enterprise.
The future of forest insect pathology research in Canada is being shaped by several drivers which are necessitating a shift in research from the chemical control paradigm to more biologically oriented approaches (Fig. 3). In addition to an accelerating global trend to reduce reliance of synthetic pesticides in favour of more natural control products, the context of forest pest management is changing from one that uses reactive “fire-fighting” approaches in response to full-blown outbreaks to using more pro-active suppression approaches earlier in outbreak cycles. Treatment with baculoviruses to suppress infestations of Douglas-fir tussock moth (Otvos and Shepherd Reference Otvos and Shepherd1991) and balsam fir sawfly (Moreau et al. Reference Moreau, Lucarotti, Kettela, Thurston, Holmes and Weaver2005) are examples of how registered pest control products can be used in an augmentative biological control approach. Other examples show that pathogens can be used successfully in traditional biological control approaches involving inoculative rather than inundative releases. Early experience with the nucleopolyhedrovirus of the European spruce sawfly (Bird and Elgee Reference Bird and Elgee1957) and the more recent example of Entomophaga maimaiga in North America and its effect on outbreak dynamics of gypsy moth (Elkinton et al. Reference Elkinton, Hajek, Boettner and Simons1991) are both (fortuitous) examples that classical biological control with pathogens can work. The most promising target pests for such an approach are established invasive exotic pests (Reference Hajek, Humber, Elkinton, May, Walsh and SilverHajek Reference Hajek, Humber, Elkinton, May, Walsh and Silverand Delalibera Reference Hajek and Delalibera2010), which have increased in importance during the past 15 years. Regulatory restrictions are making the introduction of exotic pathogens for classical biological control of introduced insect pests increasingly difficult (Lacey et al. Reference Lacey, Frutos, Kaya and Vail2001), causing a shift in focus to augmentation of indigenous pathogens as a more practical intermediate approach (e.g., Lyons et al. Reference Lyons, Lavallée, Kyei-Poku, van Frankenhuyzen, Johny and Guertin2012).
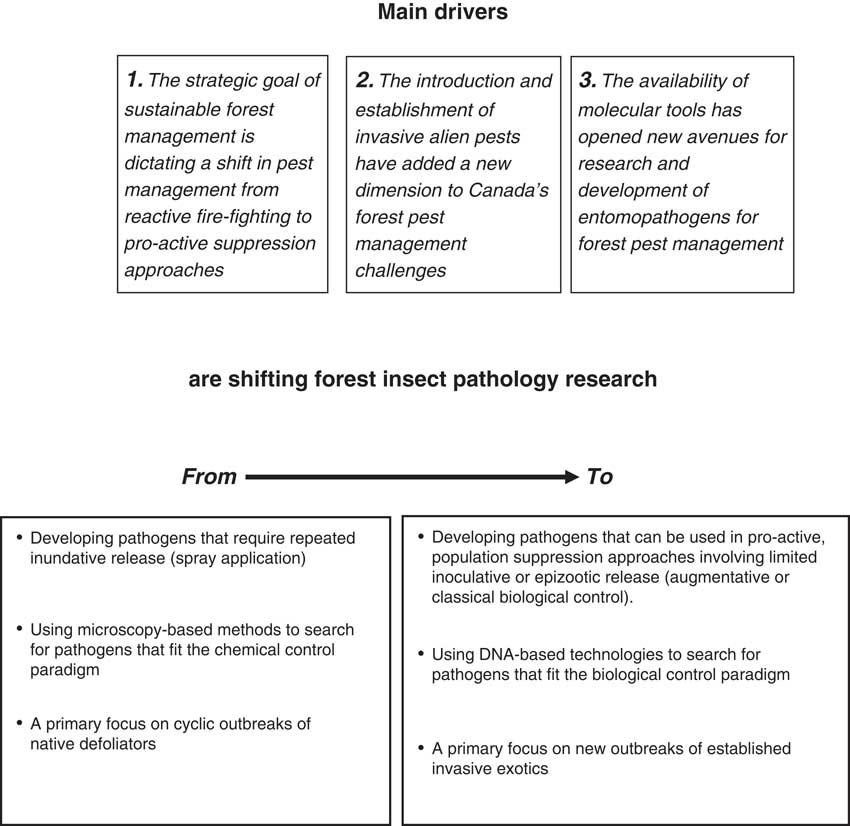
Fig. 3 Main drivers affecting the direction of forest insect pathology research.
In addition, new insights are suggesting entirely different ways of exploiting insect pathogens for pest control in the future. For example, associations of entomopathogenic fungi with host plants (Vega et al. Reference Vega, Goettel, Blackwell, Chandler, Jackson and Keller2009) involving mutualistic interactions (Behie et al. Reference Behie, Zelisko and Bidochka2012) suggest a possible role of endophytic or mycorhizal fungi that also infect insects or that produce insecticidal secondary metabolites. The latter approach is already being pioneered by J.D. Irving Ltd. in New Brunswick to obtain white spruce with high tolerance to spruce budworm feeding (Miller et al. Reference Miller, Adams and Sumarah2008; Sumarah et al. Reference Sumarah, Wilson, Miller, Slack, Adams and Berghout2008, Reference Sumarah, Blackwell, Miller, Puniani and Sorensen2010). At the same time, the advent of affordable genome sequencing and other powerful molecular tools is superseding traditional microscopy-based methods for pathogen identification and characterisation, and are offering an opportunity to re-examine natural diversity, prevalence, and ecological role of entomopathogens in forest insect populations and their habitats at an ecologically relevant scale, as a framework for the development and deployment of novel pathogen-based pest management approaches.
The shift from developing spray products to developing ecologically framed control approaches requires a new thrust of foundational research on insect pathogens and their ecology, using modern technologies. This is not in line with today’s reality of declining investments in both “public good” and basic research. Insect pathology research positions are disappearing due to natural attrition from government laboratories and universities across the country. For example, between 1965 and 1995 the Canadian Forest Service had between 10 and 15 research positions that dealt with insect pathogens in some capacity at any given time, a number that has dwindled to two or three. Similar trends are apparent at Agriculture and AgriFood Canada, the other federal department that traditionally has had significant capacity in insect pathology focussed on pests of agricultural importance. This trend clearly signals the demise of insect pathology in Canada. Unless it is reversed, the potential of insect pathogens for sustainable management of insect pests in forestry, agriculture, and public health may never be realised beyond the few spray products that have been developed to date. In that case this contribution will stand not only as the first but also as the last review of Canada’s innovations in insect pathology and the use of insect pathogens for sustainable management of forest pest insects.
Acknowledgements
The authors thank two anonymous reviewers for their constructive comments. This work was supported by the Integrated Pest Management Project of the Canadian Forestry Service, Natural Resources Canada.