Book contents
- Planetesimals
- Cambridge Planetary Science
- Planetesimals
- Copyright page
- Contents
- Contributors
- 1 Planetesimals
- Part One Dynamical Evolution
- Part Two Chemical and Mineralogical Diversity
- Part Three Asteroids as Records of Formation and Differentiation
- Part Four Early Differentiation and Consequences for Planet Formation
- Index
- References
Part Two - Chemical and Mineralogical Diversity
Published online by Cambridge University Press: 25 February 2017
- Planetesimals
- Cambridge Planetary Science
- Planetesimals
- Copyright page
- Contents
- Contributors
- 1 Planetesimals
- Part One Dynamical Evolution
- Part Two Chemical and Mineralogical Diversity
- Part Three Asteroids as Records of Formation and Differentiation
- Part Four Early Differentiation and Consequences for Planet Formation
- Index
- References
Summary
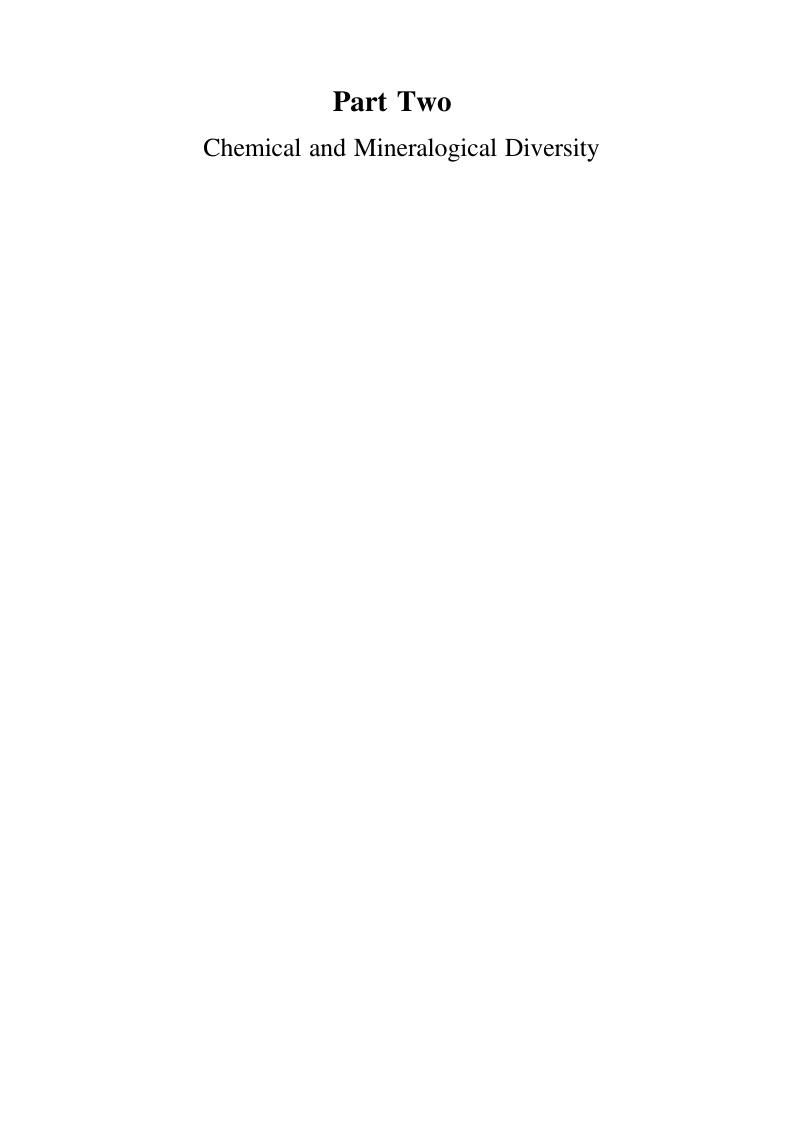
- Type
- Chapter
- Information
- PlanetesimalsEarly Differentiation and Consequences for Planets, pp. 69 - 266Publisher: Cambridge University PressPrint publication year: 2017