Book contents
- Frontmatter
- Dedication
- Contents
- Preface
- 1 Ecosystems and Climate
- Part I The Earth System
- Part II Global Physical Climatology
- Part III Hydrometeorology
- Part IV Biometeorology
- 15 Leaf Temperature and Energy Fluxes
- 16 Leaf Photosynthesis and Stomatal Conductance
- 17 Plant Canopies
- Part V Terrestrial Plant Ecology
- Part VI Terrestrial Forcings and Feedbacks
- Appendix
- Index
- Plate section
- References
16 - Leaf Photosynthesis and Stomatal Conductance
from Part IV - Biometeorology
Published online by Cambridge University Press: 05 November 2015
- Frontmatter
- Dedication
- Contents
- Preface
- 1 Ecosystems and Climate
- Part I The Earth System
- Part II Global Physical Climatology
- Part III Hydrometeorology
- Part IV Biometeorology
- 15 Leaf Temperature and Energy Fluxes
- 16 Leaf Photosynthesis and Stomatal Conductance
- 17 Plant Canopies
- Part V Terrestrial Plant Ecology
- Part VI Terrestrial Forcings and Feedbacks
- Appendix
- Index
- Plate section
- References
Summary
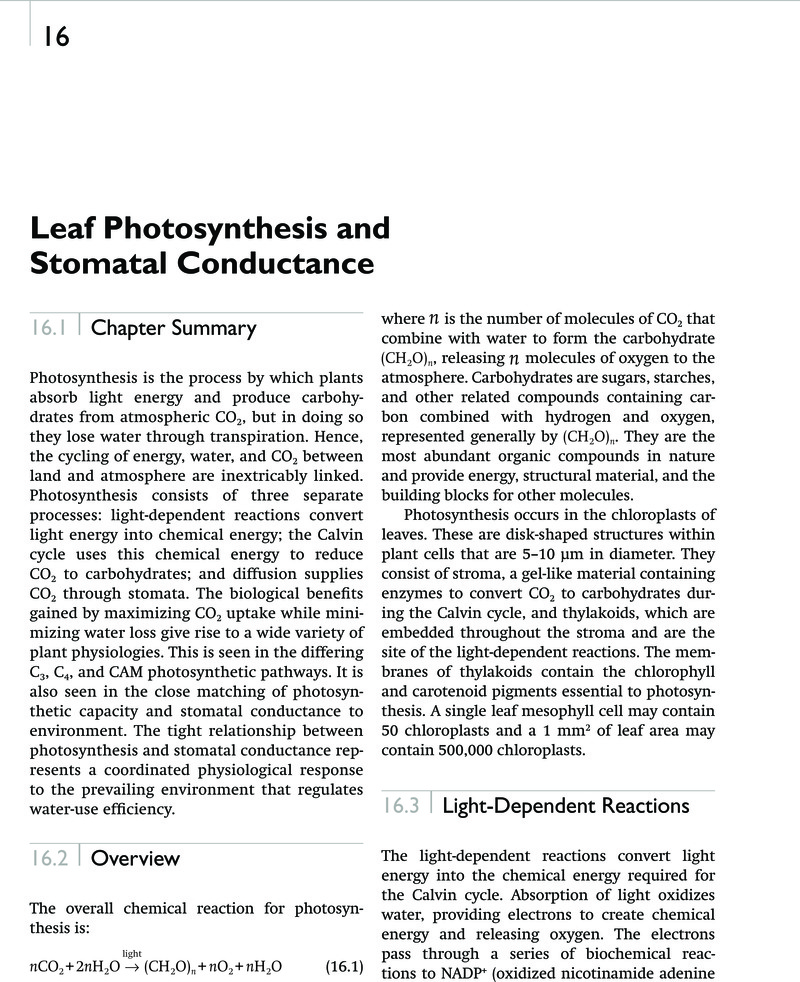
- Type
- Chapter
- Information
- Ecological ClimatologyConcepts and Applications, pp. 241 - 263Publisher: Cambridge University PressPrint publication year: 2015
References
- 3
- Cited by