The origin of vision lies far back in time, but there is still great uncertainty about how this critical event took place. There is a general consensus, however, that the origin of visual systems formed part of the so-called Cambrian Explosion; the great phenomenon that took place around 541 million years ago (Ma), when during a short stretch of time, only ca. 10–20 million years, almost all of the phyla we know today appeared. During the preceding Ediacaran, there is no unequivocal evidence of predation, thus vision was not needed for detecting predators or prey. In the lower Cambrian, animals developed harder shells, predators arose, and there began an arms race between predators and prey. The need ‘to see’ and ‘to be seen’ or ‘not to be seen’ triggered rapid evolution and helped shape the dynamics of ecological systems (Parker Reference Parker2003).
Several interesting models have been produced showing how the earliest eyes may have developed. But how, exactly, can we define what an eye actually is? Some nerve cells are highly sensitive to light, but even a specialised light-sensory cell does not, by itself, produce vision, especially if there is just one single cell. Following the definitions of Land & Nilsson (Reference Land and Nilsson2012) and Cronin et al. (Reference Cronin, Johnson, Marshall and Warrant2012), a ‘real’ eye should provide information about the contrast distribution of illuminated objects in the environment. A well-developed eye makes it possible to detect mates or food, gives information about the physical properties of the environment, enables orientation and, not least, movement discrimination, and will allow the detection of predators. Whereas this definition normally involves associated brain function from any visual input, we have to consider also box-jellyfish, which are equipped with elegant lens-eye systems, and can visually hunt fish, but have no brain or even a central ganglion.
Darwin's original idea about the origin of vision involved a single light-sensitive cell, which is shielded at one side by a pigment cell (Fig. 1a) from one original cell by unequal division of the pigment granules contained within it (Darwin Reference Darwin1859; Gehring Reference Gehring2005; Gehring & Seimiya Reference Gehring and Seimiya2010). The pigment cell shades the sensory cell from one side, so that a directional sensitivity arises. Diurnal rhythms, vertical migrations during the day, positive or negative phototaxis, and movement towards or away from a light source, as are very common in small organisms, thus becomes possible. If both cells divide several times, a small patch of light-sensitive cells and their screening partners arises, a so-called ‘eye spot’ (Fig. 1b, c). These systems are very frequent amongst invertebrates and even exist in chordates such as Branchiostoma lanceolatum (Pallas, Reference Pallas and Lange1774). They can, however, as in the original two-cell system, inform their bearers only about the presence or absence of light and its intensity. These, then, are not ‘real’ eyes, but they do provide enough information to enable the establishment of diurnal rhythms, or allow movements towards or away from light sources; in other words, phototaxis. Directionality is achieved, as soon as the eye spot is deepened to become a pit (Fig. 1d), and obliquely entering light rays are shadowed by one side of the pit. The deeper the pit, the better the direction can be distinguished. The final stage is when the pits become more or less spherical (Fig. 1e). These can then start to form inverted images – in wide open pits these images are still blurred, but the smaller the opening, the sharper the images become, but also less light reaches the small retina at the base. The ‘invention’ of a lens solves this problem. Here, a small mucous lens sits inside the pit, directly onto the retina (Fig. 1f). Systems of this kind are the so-called ocelli. Ocelli occur in many invertebrates (worms and molluscs for example), but also in arthropods as larval eyes (stemmata), or as median eyes that characterise all euarthropods. There exist several models, attempting to explain how more complex eyes developed following this stage, such as the camera eyes of humans or cephalopods, with a vitreous body that allows sharp focusing (Fig. 1g), or the compound eyes of arthropods (Fig. 1h) and polychaetes (Gehring Reference Gehring2005; Nilsson Reference Nilsson2009; Gehring & Seimiya Reference Gehring and Seimiya2010).
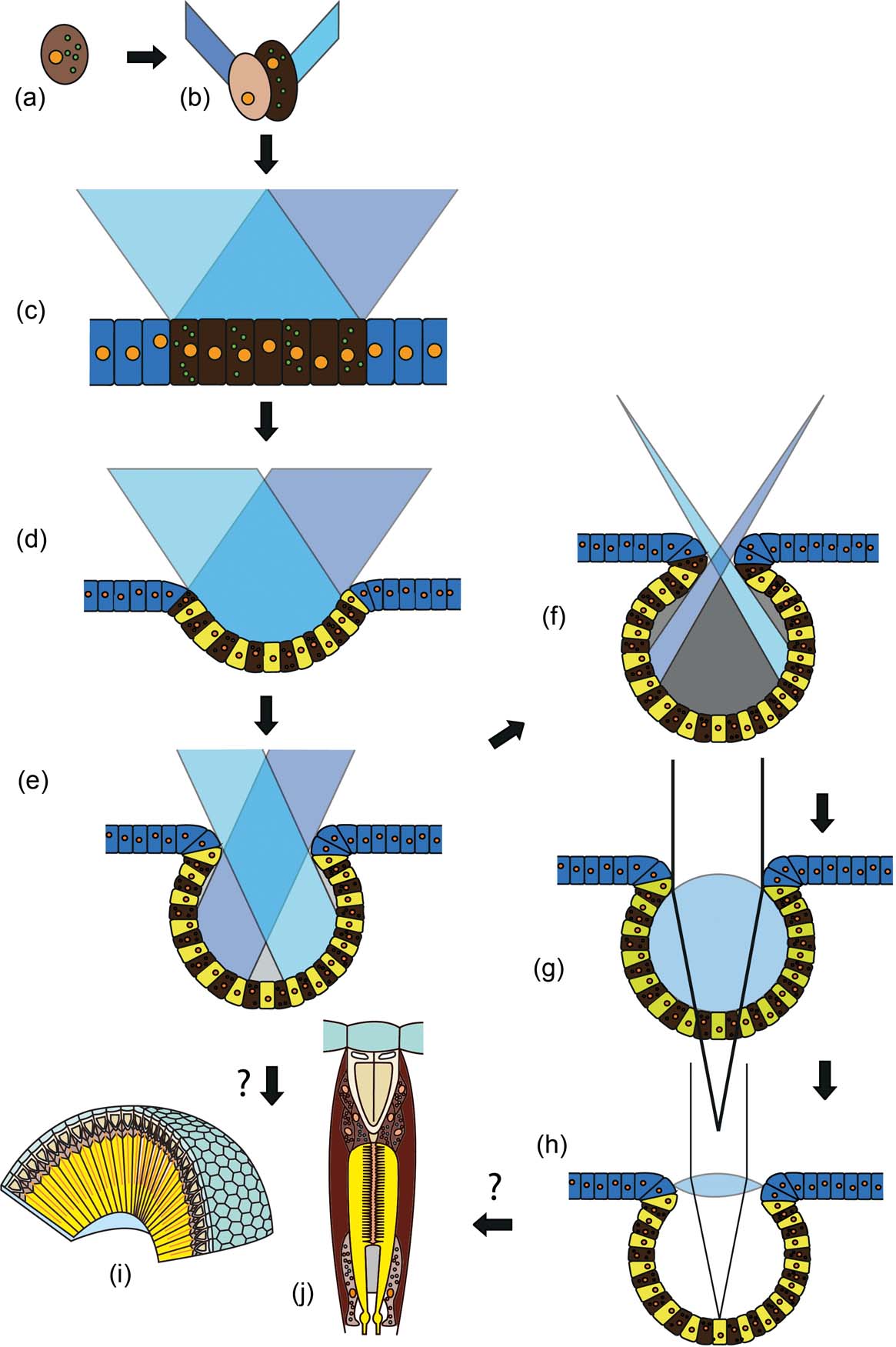
Figure 1 Origins of visual systems from a simple light-sensitive cell. For explanation see text: (a) cell with asymmetric distribution of pigments in the plasma; (b) unequal cell division, arising from a pigment and sensory cell; (c) spot eye, which cannot distinguish the direction of the incident light, but can sometimes distinguish movements, if one sensory cell after the other is shadowed by a passing object; (d) pit eye; (e) deepened pit eye (cup eye), detection of direction is possible; (f) pinhole camera eye, image formation is possible; (g) simple lens eye, under-focusing; (h) camera lens eye with high light performance; (i) compound eye (focal apposition type); (j) unit of compound eye – ommatidium (focal apposition type).
Institutional abbreviation. RCCBYU, Research Centre for the Chengjiang Biota, Yunnan University.
1. Ancient eyes in the fossil record
1.1. Cambrian arthropods
The fossil record reveals a remarkable amount of information about ancient visual systems. For example, Tanaka et al. (Reference Tanaka, Parker, Siveter, Maeda and Furutani2009) showed that superposition compound eyes were present in an Eocene fly preserved in amber, and the same authors (Tanaka et al. Reference Tanaka, Parker, Hasegawa, Siveter, Yamamoto, Miyashita, Takahashi, Ito, Wakamatsu, Mukuda, Tomokawa, Furutani, Suzuki and Maeda2014) recently found rods and cones in a 300 million-year-old (Upper Carboniferous) fish, Acanthodes bridgei Zidek, Reference Zidek1976 that are suggestive of colour. There was a great Palaeozoic diversification of different kinds of eye, especially in the Ordovician, and particularly those of trilobites. These come mainly from the early to late Cambrian (Ramsköld et al. Reference Ramsköld, Chen, Edgecombe and Zhou1997; Schoenemann & Clarkson Reference Schoenemann, Clarkson, Ahlberg and Dies-Alvarez2010, Reference Schoenemann and Clarkson2012a, Reference Schoenemann and Clarksonb, Reference Schoenemann and Clarksonc, 2015; Paterson et al. Reference Paterson, García-Bellido, Lee, Brock, Jago and Edgecombe2011; Schoenemann et al. Reference Schoenemann, Castellani, Clarkson, Haug, Maas., Haug and Waloszek2011, Reference Schoenemann, Clarkson, Castellani, Waloszek, Maas and Meyer-Rochow2014; Lee et al. Reference Lee, Torney and Owen2012). Moreover, even internal sensory structures have been described recently from the compound eyes of Devonian trilobites (Schoenemann & Clarkson Reference Schoenemann and Clarkson2013).
The organisms that appeared during the early Cambrian were already highly diversified, as also were the designs of their visual systems. Single-lens-eye systems are very clearly evident in the ocelli of lobopodians (Schoenemann et al. Reference Schoenemann, Liu, Shu, Han and Zhang2009). Their close relatives are the Recent onychophorans, and both share characteristics of arthropods and also of polychaete worms. Their eyes consist of thick lenses that sit on a cup filled with retinal and pigment cells. As is normal for ocelli, these single-lens eyes under-focus, which means that the incoming light is focused, but the image plane lies below the retinal surface, and thus the image perceived is blurred. Such an eye functions as a low-pass filter of spatial frequences, which means that only large-scale patterns of the environment can be distinguished, whilst details such as plankton or floating organic particles cannot be seen. It is easy to understand, however, that this kind of visual system provides the lobopodian with all that it needs to see – mates perhaps, physical patterns of the environment such as food, and predators moving around. Other single-lens systems may occur in early chordates, such as Haikouella lanceolata Chen, Huang & Li, Reference Chen, Huang and Li1999 or vertebrates, such as Myllokunmingia (Shu, Zhang & Han, Reference Shu, Luo, Conway Morris, Zhang, Hu, Chen, Han, Zhu, Li and Chen1999 in Shu et al. Reference Shu, Luo, Conway Morris, Zhang, Hu, Chen, Han, Zhu, Li and Chen1999), but whether their eyes are ocelli or already real camera eyes with a vitreous body, and thus a sharp imaging, awaits further research.
One of the best preserved Cambrian faunas is represented in the so-called Chengjiang Biota at multiple fossil sites close to Chengjiang in China (Hou et al. Reference Hou, Aldridge, Bergstrom, Siveter, Siveter and Feng2004). The age of this Konservat–Lagerstätte is Nangaoan, which correlates with the Atdabanian Stage of Siberia and is thus part of the traditional lower Cambrian dating around 520 Ma (Rozanov et al. Reference Rozanov, Zhu, Pak and Parkhaev2008; Zhang et al. 2008). The famous middle Cambrian Burgess Shale fauna from the Canadian Rockies consists of similar, but slightly younger forms when compared to the Chengjiang fauna, and dates back to about 505 Ma (Briggs et al. Reference Briggs, Erwin and Collier1994). The Emu Bay Shale biota (dated as Cambrian Series 2, Stage 4, about 515 Ma; Paterson et al. Reference Paterson, García-Bellido, Jago, Gehling, Lee and Edgecombe2016) closely resembles the fauna of Chengjiang, but is not as well preserved. Still under discussion is the precise correlation of these Lagerstätten with the Sirius Passet organisms (e.g., Peel & Ineson Reference Peel and Ineson2011). This location in northern Greenland is older than that of the Burgess Shale, but younger than the Chengjiang Fauna (Peng et al. Reference Peng, Babcock, Cooper, Gradstein, Ogg, Schmitz and Ogg2012); it is rather loosely dated to 518–505 Ma (Martin et al. Reference Martin, Grazhdankin, Bowring, Evans, Fedonkin and Kirschvink2000).
Almost half of the species represented in the Chengjiang Fauna consist of arthropods, and the characteristic visual systems for arthropods are compound eyes. This is true for both stem- and crown-group euarthropods, whilst the latter, as is normal, possess (four) median eyes, which are the ‘ocelli’ mentioned above. The compound eyes of the Chengjiang fauna have been the subject of a number of investigations (Schoenemann & Clarkson Reference Schoenemann, Clarkson, Ahlberg and Dies-Alvarez2010, Reference Schoenemann and Clarkson2012a, Reference Schoenemann and Clarksonb, Reference Schoenemann and Clarksonc). Amongst the Chengjiang Fauna there are two types of compound eyes. The first type shows spherical optical units, and can be found in the genera Isoxys Walcott, Reference Walcott1890, Leanchoilia Walcott, Reference Walcott1912, some anomalocaridids and others. Hexagonal facets in densely packed compound eyes (as known from modern crustaceans and insects such as dragonflies or bees) are represented, for example, in Cindarella eucalla (Ramsköld et al. Reference Ramsköld, Chen, Edgecombe and Zhou1997; Schoenemann & Clarkson Reference Schoenemann and Clarkson2012a, Reference Schoenemann and Clarksonb, Reference Schoenemann and Clarksonc; Zhao et al. Reference Zhao, Bottjer, Hu, Yin and Zhu2013). We find the same in an assumed anomalocaridid from Emu Bay (Paterson et al. Reference Paterson, García-Bellido, Lee, Brock, Jago and Edgecombe2011), an unidentified arthropod (Lee et al. Reference Lee, Jago, Garcia-Bellido, Edgecombe, Gehling and Paterson2011), and some excellently preserved crustaceans from the Upper Cambrian Orsten biota (Schoenemann et al. Reference Schoenemann, Castellani, Clarkson, Haug, Maas., Haug and Waloszek2011, Reference Schoenemann, Clarkson, Castellani, Waloszek, Maas and Meyer-Rochow2014; Parker et al. Reference Parker, Schoenemann, Haug and Waloszek2013; Schoenemann Reference Schoenemann2013).
An understanding of how these eyes functioned is possible with reference to the visual systems of living arthropods. A typical focal apposition eye can consist of a few identical units (ommatidia), or up to several thousand. Each of these consists of a corneal lens, covering a so-called crystalline cone, which consists of clear cells giving space to the incident light focused by the lens. This is collected on the tip of a light guiding structure, the rhabdom. It is part of the sensory cells, and contains the visual pigments. The incident light changes the steric conformation of these pigments, which evokes an electrical signal that can be processed by the nervous system and results in ‘vision’. Because the contrasts inside the visual field of each ommatidium are combined onto the tip of the rhabdom, and because all individual ommatidia are isolated against their neighbours by pigment cells, over the total compound eye a mosaic-like image of vision is formed. The resolution of this vision is partly related to the number of ommatidia, likened to the number of pixels in a computer graphic. Nowadays, this system is found abundantly in modern diurnal crustaceans and insects, namely in dragonflies or bees.
In the earliest upper Cambrian Alum Shales of Sweden, ‘Orsten’ limestone nodules can be found dated to the Agnostus pisiformis Biozone (for an overview see Müller Reference Müller1983; Müller & Walossek Reference Müller and Walossek1985). They contain phosphatised, three-dimensionally preserved crustaceans and other fossilised organisms that present finest details, such as bristles and compound eyes. Analysis of these eyes has shown that these early crustaceans possessed focal apposition eyes (Schoenemann Reference Schoenemann2013; Schoenemann et al. Reference Schoenemann, Clarkson, Castellani, Waloszek, Maas and Meyer-Rochow2014), with a structure comparable to that of modern diurnal arthropods such as bees. Parker et al. (Reference Parker, Schoenemann, Haug and Waloszek2013) were able to show that amongst these crustaceans, a sophisticated yellow cornea had been developed, filtering blue scattering light and enhancing the contrast underwater. Finally, the concept of an effective arrangement of facets in a compound eye, which was too small to supply an image, but able to detect small objects in a kind of sophisticated three-dimensional coordinate system (Schoenemann et al. Reference Schoenemann, Castellani, Clarkson, Haug, Maas., Haug and Waloszek2011), has given clear insights into how elegant and sophisticated the visual systems of crustaceans had already become during Cambrian times.
In aquatic systems, the difference between the refractive indexes of water and the organic material is so low that the thin corneal lens has hardly any refractive power, and in modern systems the crystalline cone often takes over the refraction by forming cylinders with index gradients. Lenses with the highest refractive power are spherical, and this may be the reason the dioptric elements are globular in the Cambrian arthropod Isoxys and others (Fig. 2).
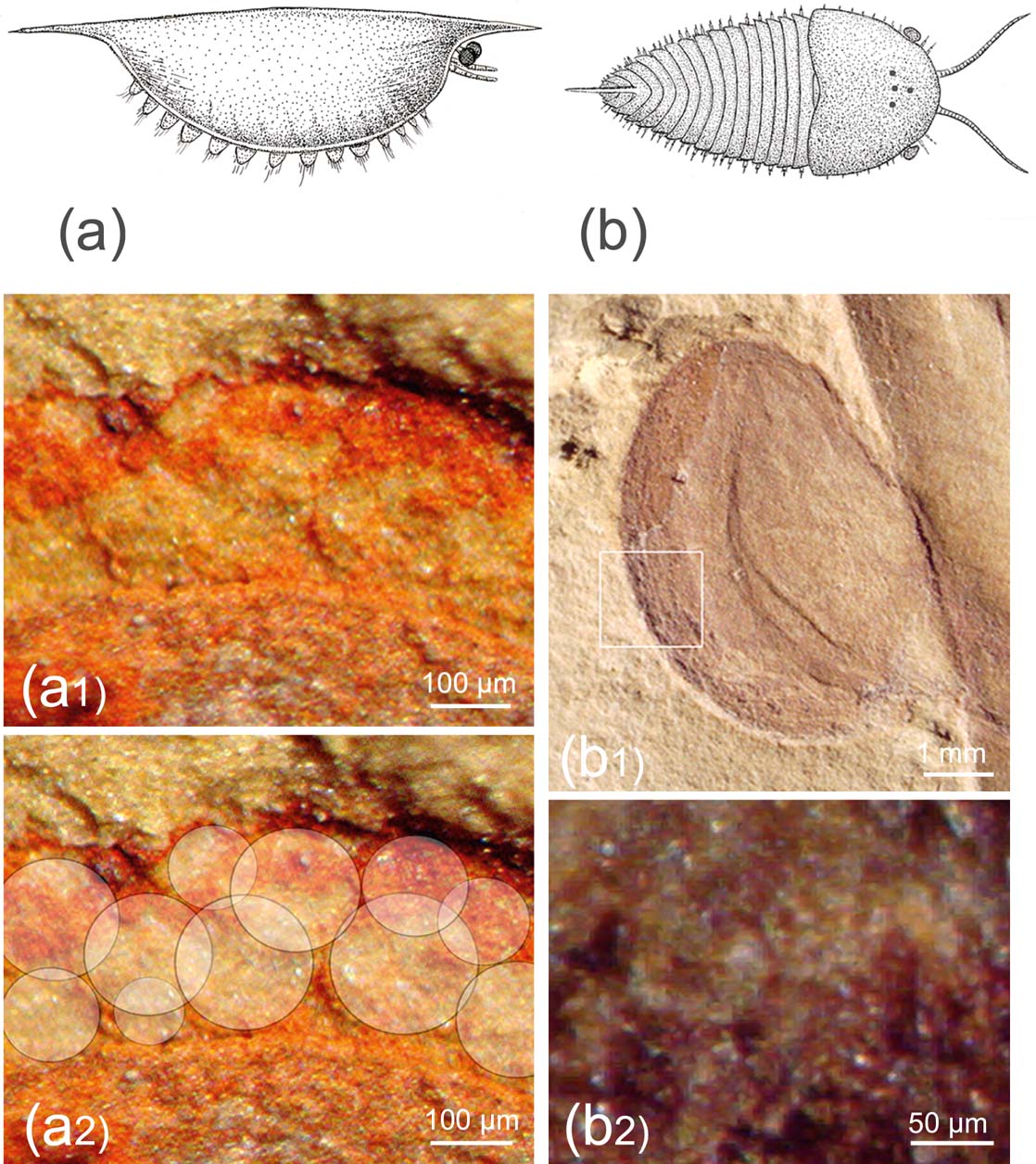
Figure 2 Vision in Cambrian arthropods. (a, a1, a2) Isoxys auritus (Jiang, Reference Jiang, Luo, Jiang, Wu, Song and Ouyang1982): (a) reconstruction, based on Vannier & Chen (Reference Vannier and Chen2000) and Hou et al. (Reference Hou, Aldridge, Bergstrom, Siveter, Siveter and Feng2004); (a1) compound eye, specimen RCCBYU 10262, Maotianshan, Kunming, China, Lower Cambrian; (a2) visual units of a1 marked. (b, b1, b2) Cindarella eucalla Chen, Ramsköld et al., Reference Ramsköld, Chen, Edgecombe and Zhou1997: (b) reconstruction based on Ramsköld et al. (Reference Ramsköld, Chen, Edgecombe and Zhou1997); (b1) compound eye, specimen RCCBYU 10288, Maotianshan Shale Member at Mafang, Haikou; (b2) individual facets from b1.
The elements of trilobite eyes seen in Figure 3 are almost-spherical lenses. Similar systems in which the crystalline cone, functioning as a refractive unit, appears almost spherical are found in many modern crustacean eyes of today, such as those of isopodes or many amphipodes. The more advanced systems seem to be densely packed with the hexagonal facets mentioned above. Optimal dense packing of originally spherical systems results in hexagons, as we know, for example, in the combs of honey bees. Although the high number of facets leads to an enhanced acuity, the small lens-apertures impose constraints on visual function, because of the lower number of photons that can reach the sensory cells. The internal structure of these fossilised compound eyes, however, remains as yet unknown. Median eyes, which are a synapomorphy of all euarthropods, can be found in bearers of both systems (e.g., Cindarella and Leanchoilia; Fig. 2), and it seems clear that the same systems, whether equipped with spherical optical elements or as compound eyes with densely packed hexagonal facets, are still present in modern and advanced organisms, still living today.
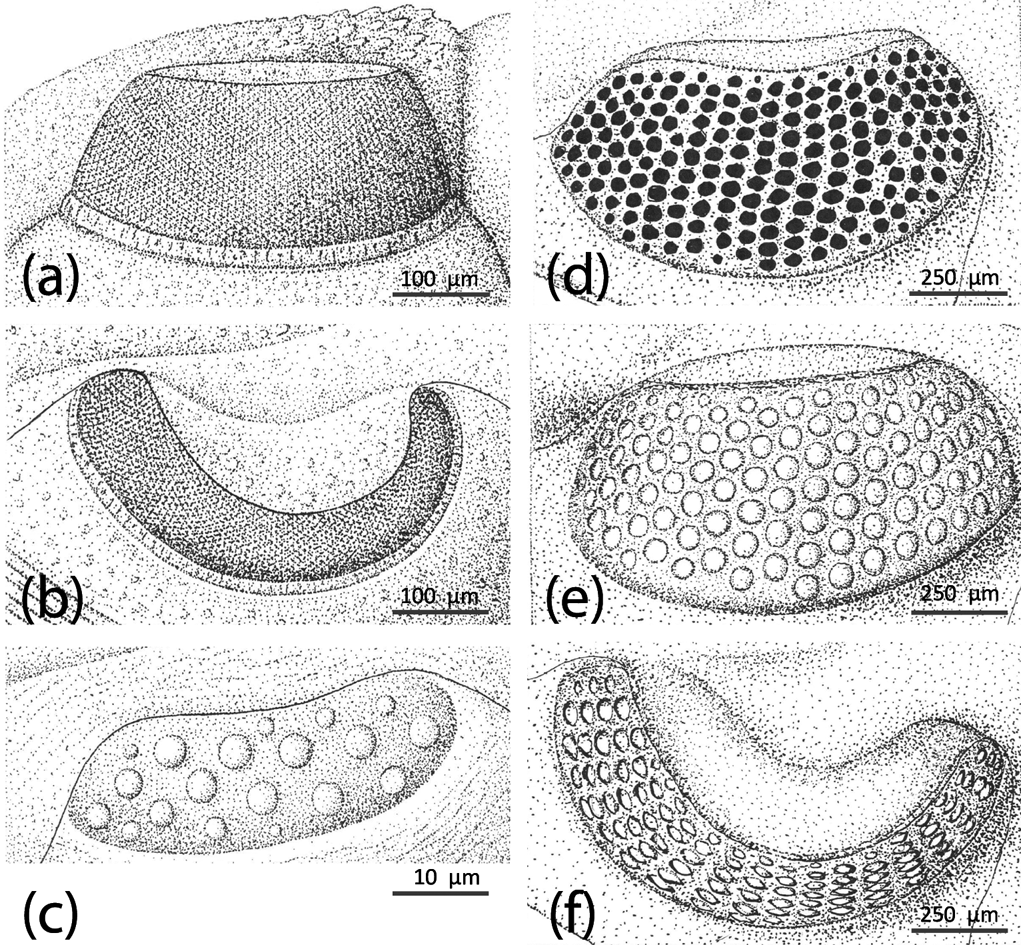
Figure 3 Holochroal and schizochroal eyes. (a–c) Holochroal eyes of Paladin eichwaldi shunnerensis (King, Reference King1914), middle Carboniferous, Yorkshire, England. Left holochroal eye of an adult in (a) lateral (b) dorsal views; (c) juvenile eye of a degree 0 meraspis. All based on Clarkson & Zhang Reference Clarkson and Zhang1991. (d) Adult schizochroal eye of Ormathops atavus (Barrande, 1872), early Ordovician, showing irregularities in lens packing, internal mould. Based on Clarkson (Reference Clarkson1971). (e–f) Adult schizochroal eye of Eophacops trapeziceps (Barrande, Reference Barrande1846), Silurian, Bohemia, in (e) lateral and (f) dorsal views. Based on Thomas Reference Thomas1998.
Physiologists such as Snyder, Land, Nilsson and others have developed physical tools for describing the light ecological adaptations of living arthropods (Horridge Reference Horridge1977, Reference Horridge1978; Snyder Reference Snyder1977, Reference Snyder and Autrum1979; Snyder et al. Reference Snyder, Stavenga and Laughlin1979), because the geometry of their compound eyes allow conclusions about how they process the incident light. Many of these tools can be applied, sometimes in a modified form, to fossils, and this allows an assignment to their ecological habitat and, in particular, the relative depth of the ocean they inhabited. This has been undertaken for the Chengjiang fauna (Schoenemann & Clarkson Reference Schoenemann, Clarkson, Ahlberg and Dies-Alvarez2010, Reference Schoenemann, Castellani, Clarkson, Haug, Maas., Haug and Waloszek2011, Reference Schoenemann and Clarkson2012a, Reference Schoenemann and Clarksonb, Reference Schoenemann and Clarksonc) and Emu Bay eyes (Reference Lee, Jago, Garcia-Bellido, Edgecombe, Gehling and PatersonLee et al. 2011; Paterson et al. Reference Paterson, García-Bellido, Lee, Brock, Jago and Edgecombe2011), and also for the excellently preserved arthropods of Bundenbach (Lower Devonian, Hunsrück Shale), which could be assigned to waters up to 200 m depth (Rust et al. Reference Rust, Bergmann, Bartels, Schoenemann, Sedlmeier and Kühl2016), as well as for eurypterids (Anderson et al. Reference Anderson, McCoy, McNamara and Briggs2014; Poschmann et al. Reference Poschmann, Schoenemann and McCoy2016) and for a variety of trilobites (Fordyce & Cronin Reference Fordyce and Cronin1989, Reference Fordyce and Cronin1993; McCormick & Fortey Reference McCormick and Fortey1998; Schoenemann et al. Reference Schoenemann, Clarkson, Franz, Rábano, Gozalo Gutiérrez and García-Bellido2008a, Reference Schoenemann, Clarkson, Ahlberg and Dies-Alvarez2010; Schoenemann & Clarkson Reference Schoenemann and Clarkson2015; Tanaka et al. Reference Tanaka, Schoenemann, El Hariri, Ono, Clarkson and Maeda2015). Other visual systems in the fossil record, such as those of the Carboniferous shrimps Tealliocaris (Briggs & Clarkson Reference Briggs and Clarkson1985), remain to be investigated.
1.2. Trilobites
Trilobites are extinct marine arthropods that dominated the Palaeozoic. The earliest trilobites appeared, however, at the base of the Atdabanian (Cambrian Series 2, Stage 3; ca 521 Ma).
Following the early work of Clarke (Reference Clarke1889) and Lindström (Reference Lindström1901) on the structure of trilobite eyes, much has been written about them since the 1960s, and various summaries have been presented (e.g., Campbell Reference Campbell1975; Clarkson Reference Clarkson1975, Reference Clarkson, Moore and Kaesler1997; Levi-Setti et al. Reference Levi-Setti, Clarkson and Horvath1998; Clarkson et al. Reference Clarkson, Levi-Setti and Horvath2006, Reference Clarkson, Levi-Setti and Horvath2008; Levi-Setti Reference Levi-Setti2014). Here, our intention is to give an account of recent developments, especially in our understanding of the structure and functioning of these eyes.
1.2.1. A brief history
The earliest of all trilobites from the lowermost Cambrian Series 2 (c. 521 Ma) were equipped with compound eyes on their first appearance in the fossil record, as were the great majority of trilobites throughout the whole time span of this group. Indeed, the very last trilobites from the end Permian (c. 252 Ma), (Clarkson Reference Clarkson1998; Fortey & Owens 1977) had eyes not externally dissimilar to those of the early Cambrian trilobites (Feist & Clarkson Reference Feist and Clarkson1989). Whereas the origin of trilobites remains mysterious, it is well known that, very early in their history, these marine arthropods rapidly spread all over the world and colonised many environments, in deep and shallow waters of the continental shelves (Clarkson Reference Clarkson1998). There was a continuous increase of trilobite genera and species throughout the Cambrian, though many of them remained benthic (Webster Reference Webster2007). Following a major crisis at the end of the Cambrian, trilobites recovered and quickly diversified, colonising various environments including reef, pelagic and deep-water habitats (Clarkson Reference Clarkson1998; Rudkin et al. Reference Rudkin, Young, Elias and Dobrzanske2003). The Ordovician was the acme of the trilobites, a time when they diversified more extensively than at any other time in geological history, with the greatest degree of morphological disparity (Adrain et al. Reference Adrain, Fortey and Westrop1998), and there was also a corresponding expansion in the number of eye types. During the latest Ordovician, the short-lived but severe Hirnantian glaciation had serious effects on the trilobites (Adrain et al. Reference Adrain, Fortey and Westrop1998), as it did on most other marine invertebrates (Sheehan Reference Sheehan2001). Many morphotypes disappeared for ever, and some ecological niches, such as the pelagic realm, lost many typical trilobite inhabitants. Moreover, no radically new body plans originated after the early Ordovician, and trilobite evolution thereafter was no more than a matter of variation on themes established at that time.
The Silurian and Devonian history of the trilobites, though they still remained abundant and diversified in some environments, was a case of a long slow decline (Fortey & Owens 1977). The Silurian faunas are basically an impoverished relic of types established in the early Ordovician. Those of the Devonian are rich and diversified in some parts of the world, such as Morocco (Chatterton et al. Reference Chatterton, Fortey, Brett, Gibb and Kellar2006; McKellar & Chatterton Reference McKellar and Chatterton2009) and Algeria (Crônier et al. Reference Crônier, Malti, François, Benyoucef and Brice2013; Khaldi et al. Reference Khaldi, Crônier, Hainaut, Abbache and Ouali Mehadji2016) and, indeed, there were some distinctive adaptive radiations (Crônier & Courville Reference Crônier and Courville2003; Crônier & François Reference Crônier and François2014). Otherwise, it was a matter of stepwise extinction of one group after another. The late Devonian trilobite crises (Feist Reference Feist1991; Clarkson Reference Clarkson and McLeod2013) disposed of all remaining groups, save for those of the Order Proetida, which continued throughout the whole of the Carboniferous and Permian until their final extinction over 90 million years later. During this time, they remained relatively small and had a conservative morphology, with the various genera not greatly different from one another. In addition, they were restricted to relatively few habitats, but to these they were well adapted. The great end-Permian crisis finally terminated a history of some 270 million years.
1.2.2. Holochroal eyes
It has always been recognised that there are two main types of compound eye in trilobites, with some possibility of a third type (Zhang & Clarkson 1990, 1993, 2012). Holochroal eyes are apomorphic for trilobites and are the primary kind from which all variations were derived (Clarkson et al. Reference Clarkson, Levi-Setti and Horvath2006). These eyes are first found in the lower Cambrian, but persist in various manifestations until the final extinction of the trilobites at the end of the Permian. Holochroal eyes (Fig. 3a–c) are typically kidney-shaped, and often have two components. First there is the lens array, which consists of many small contiguous lenses of calcite, forming a curving visual surface which surveys a substantial field of view. Secondly, the visual surface is often typically set on a socle at the base (Schoenemann et al. Reference Schoenemann, Clarkson, Ahlberg and Dies-Alvarez2010). The basic structure of the holochroal eye was remarkably conservative; the earliest Cambrian eyes are not externally dissimilar from those of the Permo–Carboniferous.
Evolution in holochroal eyes was very much a matter of permutations on a basic theme established during the lower Cambrian. These variations include the size of eye, its shape, the number of lenses and their diameter and convexity. Trilobites with thick shells, such as the asaphids, tend to have long cylindrical lenses, whereas in olenids and other ‘thin-shelled’ trilobites, the lenses are slim and usually biconvex (Clarkson Reference Clarkson1979). In all trilobite lenses, the use of simple Gaussian formulae enables the focal length of the lenses to be established (Clarkson Reference Clarkson1979). In general terms, the relative curvature of the surfaces ensures that the focal point lies at about the same general distance below the base of the eye. The largest eyes are found in Ordovician pelagic trilobites, such as Opipeuterella, Telephina and Cyclopyge (Fortey Reference Fortey1985, Reference Fortey2000). In these forms, the two eyes may be fused anteriorly, and the visual field may be panoramic, extending ventrally as well as laterally and dorsally.
It may be assumed that below each lens in the holochroal eye there lies an ommatidium-like unit; the main difference between the eyes of a crustacean and those of a trilobite is that the crustacean lenses are organic, whereas trilobite lenses are composed of calcite. A current investigation of new material of some lower Cambrian trilobites is revealing sublensar structures for the first time.
1.2.3. New developments in the study of holochroal eyes
Magnificent new colour illustrations by Levi-Setti (Reference Levi-Setti2014) serve to show the range in form of trilobite eyes, and of these, the amazing stalked holochroal eyes of some asaphids from the St Petersburg region (Wolchow river area) are especially noteworthy. It is well known that in most post-Cambrian trilobites, though not all, the visual surface is retained after death or moulting, being firmly attached to the eye socle. For most Cambrian trilobites (except for some of the olenids), however, this is not the case. This is because an additional suture is present, the ocular suture, which runs along the lower edge of the visual surface. This joins with the palpebral suture, so that in the adult a suture encircles the eye (forming the circumocular suture), which allows the visual surface to drop out from a dead animal or its moulted exuviae. It remains attached, however, in the meraspides, though these are not often preserved. A well-preserved specimen of Eoredlichia intermediata (Lu, Reference Lu1940), from the Chengjiang Biota, retains the adult eye, with at least 30 lenses, forming a single line close to the palpebral suture (X.–g. Hou, pers. comm. 2014). The rest of the eye is damaged and the corneal membrane has been dissolved. This unusual specimen confirms that the earliest known trilobite eyes were typically holochroal.
Other recent studies have involved the use of the eye parameter to determine the relative depths at which different trilobites lived (McCormick & Fortey Reference McCormick and Fortey1998; Schoenemann et al. Reference Schoenemann, Clarkson, Franz, Rábano, Gozalo Gutiérrez and García-Bellido2008a, Reference Schoenemann, Clarkson, Ahlberg and Dies-Alvarez2010; Schoenemann & Clarkson Reference Schoenemann and Clarkson2015; Tanaka et al. Reference Tanaka, Schoenemann, El Hariri, Ono, Clarkson and Maeda2015).
In any compound eye, the visual surface is curved, so that each ommatidium points in a slightly different direction to its neighbours. The angle between the optical axes of two adjacent ommatidia, the interommatidial angle, is known as Δφ. For high acuity (i.e., maximum resolution and sharpness of the image), Δφ needs to be as low as possible. But acuity also depends upon the number of lenses, and there are only limited numbers of these that can be accommodated on the visual surface, depending on the diameter of each ommatidium. The smaller the lenses, the fewer photons they can capture and, as such, are poorly adapted to dimly-lit environments. They must have a minimal diameter, defined as D. There is inevitably a trade-off between the requirements of light gathering (wide lenses, high D) and visual acuity (small lenses, low Δφ). These conflicting requirements are resolved in different ways by arthropods inhabiting different environments, and their eyes are optimised for their specific ‘light-ecological’ habitat. This can readily be determined, at least in relative terms, and even in fossil arthropods, by the eye parameter D · Δφ, measured in μ rad.
Thus measurement of the diameter D of lenses, and the curvature of the visual surface and the number of lenses upon it, or any other measurements to establish Δφ, will suffice to provide the necessary information. Using the eye parameter, McCormick & Fortey (Reference McCormick and Fortey1998) and Tanaka et al. (Reference Tanaka, Schoenemann, El Hariri, Ono, Clarkson and Maeda2015) were able to show that two pelagic large-eyed cyclopygids swam at different depths in the ocean. Likewise, Schoenemann et al. (Reference Schoenemann, Clarkson, Franz, Rábano, Gozalo Gutiérrez and García-Bellido2008a, Reference Schoenemann, Clarkson, Ahlberg and Dies-Alvarez2010) established that the tiny olenid Ctenopyge ceciliae Clarkson & Ahlberg, Reference Clarkson and Ahlberg2002 was an inhabitant of well-illuminated shallow waters, and that the spiny olenids Sphaerophthalmus alatus (Boeck, Reference Boeck and Keilhau1938) and Ctenopyge (Mesoctenopyge) tumida Westergård, Reference Westergård1922, though found together in the same beds, were adapted to different light-ecological habitats (Schoenemann & Clarkson Reference Schoenemann and Clarkson2015); the former living in a dimly-lit environment (most likely on the sea floor), the latter in a well-illuminated habitat (likely as a pelagic swimmer).
1.2.4. Schizochroal eyes
During the 270 million years of trilobite evolution, there is only one clade that made a radical, but evidently successful, departure from the holochroal system. This is the schizochroal eye (Fig. 3d–f), confined to the Lower Ordovician to Upper Devonian Suborder Phacopina, which persisted for about 130 Ma. In these eyes, the lenses are much larger and fewer than those of holochroal eyes, and they are separated one from another by ‘interlensar sclera’, effectively the same material as the rest of the exoskeleton (Clarkson Reference Clarkson1967, Reference Clarkson, Moore and Kaesler1997). The lens array surveys a visual field which is directed laterally, forwards and backwards, but never overhead. The large lenses have been shown to have a complex internal structure (Lindström Reference Lindström1901; Clarkson & Levi-Setti Reference Clarkson and Levi-Setti1975; Lee et al. Reference Lee, Torney and Owen2012; Torney et al. Reference Torney, Lee and Owen2014). Much attention has been given to the functioning of the schizochroal eye, which has no close resemblance to any other kind of visual organ in the whole of the animal kingdom (Reference Horváth, Clarkson and PixHorvath et al. 1997).
1.2.5. Origins of the schizochroal eye revisited
Trilobites grew by successive moults, and the discarded exuviae, and rare cadavers preserved in the fossil record, can be arranged in a gradational size series for many species, showing the whole course of ontogeny from larva to adult. In some exceptionally well-preserved meraspides of species that have holochroal eyes in the adult form, the tiny visual systems are preserved in fine detail. In several cases, notably the Cambrian Olenus (Clarkson & Taylor Reference Clarkson and Taylor1995) and the Carboniferous Paladin (Clarkson & Zhang, Reference Clarkson and Zhang1991) (Fig. 3a–c) the lenses are few, separated from one another and relatively large for the size of the visual surface. In other words, such an eye looks more like a schizochroal eye than an adult holochroal eye. This seems to have been the general trend for all holochroal eyes. In considering the origin of the schizochroal eye, the most likely first evolutionary step would have been via paedomorphosis, a simple mutation leading to the retention of the ancestral juvenile structure (or state) into the adult of the descendant. This appears to have happened by the early Ordovician in phacopids, which possess the first schizochroal eyes, though the origin of this group remains a classic case of cryptogenesis.
The origin of schizochroal eyes in early Ordovician phacopids is interesting in this regard. If their eyes developed as a result of paedomorphosis, they inherited from their holochroal ancestors a visual surface with relatively few, separated lenses that are large relative to the surface of the eye, but are all more-or-less the same size. It is known that in all trilobite eyes, whether holochroal or schizochroal, the visual surface grows forwards and downwards. Thomas (Reference Thomas1998, Reference Thomas2005) proposed a model for how this may have happened, based on the living Drosophila. Here, during eye-formation, a ‘morphogenetic furrow’ sweeps across the imaginal disc, generating a ‘wave’ of lens emplacement running across the eye. Quite possibly, this is an ancient, conserved developmental programme, and may be common to all arthropods. The first row of (small) lenses in trilobites is emplaced just below the palpebral suture. This is most easily seen in the phacopids. Whilst the eye remains tiny there are only a few lenses, and these are preserved as the accessory upper horizontal row. In some of the large-eyed dalmanitids, there may actually be more than one such accessory row. Thus, lenses emplaced in alternate rows below existing lenses leads to a clear pattern of hexagonal close packing. But in some of the early Ordovician genera, such as Ormathops atavus (Barrande, 1872) (Fig. 3d) and Toletanaspis, the regularity of lens packing breaks down. Several examples of this were shown by Clarkson (Reference Clarkson1971); in some instances, within the same species, separate blocks of parallel files of identical-sized lenses are separated by discontinuities (caesurae), whilst in others, parts of the visual surface have the lenses arranged in an irregular and haphazard system. The packing arrangement is seldom the same in members of the same population, or even in the two eyes of a single individual. There is a good reason for this. The critical issue here is that the genetic programme for the growth of the visual surface is decoupled from that for lens emplacement. The downward expansion of the visual surface with growth, which would accommodate more lenses, conflicts with the emplacement of the lenses along dorso-ventral vectors. The result is an irregular emplacement. But in later phacopids, regularity is ensured by a small but regular downward increase in the spacing of the lens centres and a concomitant enlargement of the size of the lenses. This surely tells us that regularity of packing is important for the vision of schizochroal eyes, and this would accord well with the neural superposition scheme proposed by Schoenemann (Reference Schoenemann2007). The irregular packing of the lenses in the Ormathops complex is one of the few imperfect adaptations that we see in trilobites.
1.2.6. New developments in the interpretation of schizochroal lenses
It is well established that (i) the original material of which all trilobite lenses are constructed is orientated calcite (Towe Reference Towe1973; Clarkson Reference Clarkson, Moore and Kaesler1997; Clarkson et al. Reference Clarkson, Levi-Setti and Horvath2006); (ii) the lenses of schizochroal eyes are internally differentiated into three separate components, the upper unit, the intralensar bowl, and the core (Clarkson Reference Clarkson1967, Reference Clarkson1969; Clarkson & Levi-Setti Reference Clarkson and Levi-Setti1975; Miller & Clarkson Reference Miller and Clarkson1980; Lee et al. Reference Lee, Torney and Owen2012; Torney et al. Reference Torney, Lee and Owen2014). The upper lens unit and the intralensar bowl form a doublet system with an aplanatic interface, bringing light to a sharp focus; and (iii) all trilobite eye lenses consist of slender, orientated calcitic microcrystallites known as trabeculae, which traverse the lens from top to bottom. That the calcite may show fluorescence which, however, is not of biological relevance, has been discussed recently (Schoenemann et. al. Reference Schoenemann, Clarkson and Horváth2015).
Bruton & Haas (Reference Bruton and Haas2003) believed that all internal structures that had been recorded within the eyes of trilobites were artefacts. They proposed instead an interesting alternative theoretical concept, the Graded Refractive Index model. But the fact that the internal structures they illustrated are remarkably similar in each lens and, moreover, closely resembled those already described, did not lead to a general acceptance of the new hypothesis. Further proof of the primary nature of the upper unit, bowl and core came from the detailed mineralogical analyses of Lee et al. (Reference Lee, Torney and Owen2007) and Torney et al. (Reference Torney, Lee and Owen2014). They used various cutting-edge techniques, which gave an understanding of the structure of the calcitic lenses in detail never seen before. Although these authors acknowledge that these internal structures have undergone diagenetic alteration, they consider that there were indeed original differences in mineral chemistry between the upper lens unit and the bowl. Both the bowl and the core (which is primary) contain pervasive micropores and microdolomite inclusions, both products of replacement of original magnesian calcite. The bowl and core (but especially the former) were constructed of high magnesian calcite; in contrast with the upper lens unit, in which the magnesium content was lower. These studies confirm that there was indeed a chemical contrast between the upper unit and the other components, thus sustaining the doublet hypothesis. The rest of the trilobite exoskeleton is made of low-magnesian calcite, and the presence of high-magnesian calcite in the bowl and core clearly indicates a simultaneous crystallisation of both low and high magnesian calcite (Lee et al. Reference Lee, Torney and Owen2007; Torney et al. Reference Torney, Lee and Owen2014). These authors also note that such “a strong biological control on the chemical composition of calcite indicates that magnesium was necessary for the functioning of the optical system, most probably through its influence on the refractive index of calcite” (Lee et al. Reference Lee, Torney and Owen2012, p.1036). The optical functions of various components of the lenses have been investigated by Horvath and his colleagues; the intralensar bowl reduces reflectivity (Horvath Reference Horváth1996), and the core appears to have been involved in image formation (Egri & Horvath Reference Egri and Horvath2012).
A further development was proposed by Schoenemann & Clarkson (Reference Clarkson, Levi-Setti and Horvath2008, Reference Schoenemann and Clarkson2011). Although the classic model of Clarkson & Levi-Setti (Reference Clarkson and Levi-Setti1975) holds for the majority of trilobites, there are others in which there appear to be gaps between adjacent trabeculae. If these, in life, had been filled with organic matter, then each trabeculum would have been isolated from its neighbours. A simple model was proposed for the generation of the organic sheath. If the trabecula had indeed been isolated in this way, then, as a result of total internal reflection, they would act as light guides, and thus the whole lens could act as a light-guide bundle. The result would be a pixelated visual system, unknown elsewhere in the animal kingdom, but with evident parallels with modern optical technology.
Torney et al. (Reference Torney, Lee and Owen2014) have proposed an elegant new model for the growth of the lenses. Distinguishing between low-convexity (dalmanitid) and high-convexity (phacopine) lenses, their detailed analysis shows that the (often fan-like) trabeculae do not quite extend to the outer surface of the lens, where instead there is a radial fringe of very thin calcite sheets, either annular (Figs 4b, 5), or extending across the eye, whose c-axes lie at a low angle to the surface. In high-convexity lenses, the radial fringe is thicker, and the trabeculae seem to be absent. Both kinds of lenses are regarded as having formed as a result of a migrating surface moving downwards from the cornea. Whether this concept bears upon the light-guide model remains debatable; we do not believe that it invalidates our model. But it illustrates the extraordinary complexity of the schizochroal eye and how much has already been revealed by modern technology; but also how much more remains to be done.
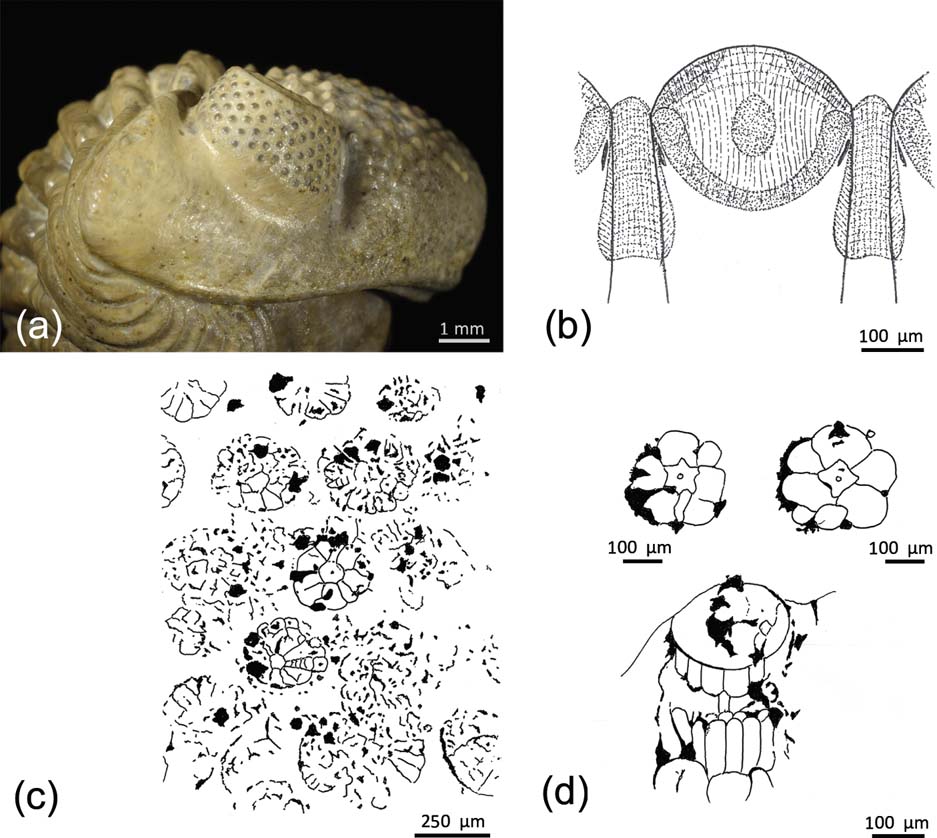
Figure 4 (a) Right schizochroal eye of an enrolled specimen of Geesops schlotheimi (Bronn, Reference Bronn1825), Middle Devonian, Gees-Gerolstein, Germany, showing lenses. (b) Vertical section through a lens of a schizochroal-eyed trilobite, with the capsule below, modified from Miller & Clarkson (Reference Miller and Clarkson1980), with additions from Torney et al. (Reference Torney, Lee and Owen2014). (c, d) Sublensar structures in schizochroal eyes, redrawn from Schoenemann & Clarkson (Reference Clarkson and McLeod2013), revealing the original contents of capsules: (c) cross-section through the upper third of compound eye, showing preservation through mineral seeding on slightly rotted and disturbed original structures; (d) two well-preserved visual units in cross-section and vertical section through a visual unit, lying within the capsule. Redrawn from Schoenemann & Clarkson (Reference Clarkson and McLeod2013).
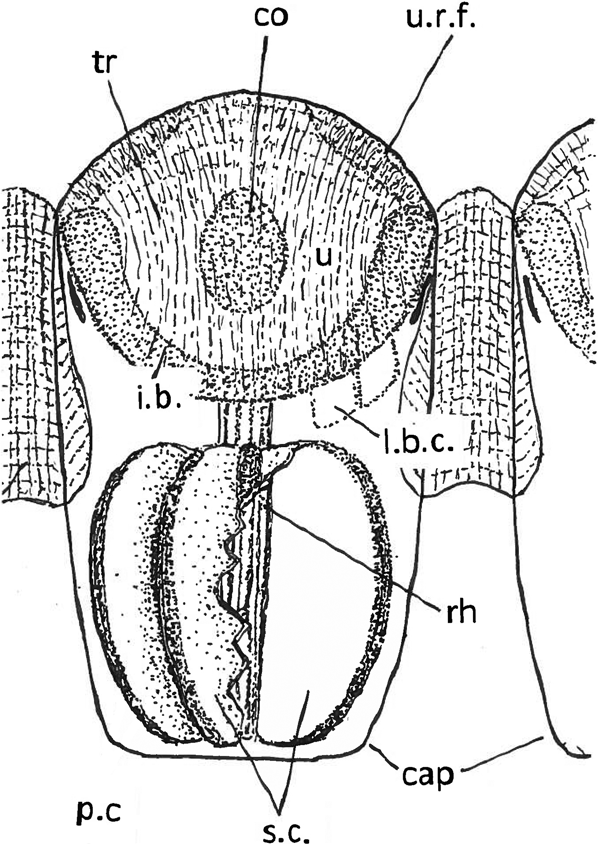
Figure 5 Schematic reconstruction of a lens of a schizochroal phacopid eye lens and capsule with contents. Lens modified from Miller & Clarkson (Reference Miller and Clarkson1980), with additions from Torney et al. (Reference Torney, Lee and Owen2014). Contents of the capsule tentative, based on Schoenemann & Clarkson (Reference Clarkson and McLeod2013); see also Fig. 4d. Rhabdom covers (pigment?) cells and sensory cells. The positions of two of the lens-building (?) cells are shown on the right hand side. The sensory elements are shown cut away on the right hand side to show the flat inner face of a sensory cell; the left side is intact. Abbreviations: cap = capsule; co = core; i.b. = intralensar bowl; l.b.c. = lens-building (?) cells; p.c = pigment (?) cells; rh = rhabdom; sc = sensory cells; tr = trabeculae; u = upper lens-unit; u.r.f. = upper radial fringe.
1.2.7. Sublensar structures in phacopid eyes
A significant development in our understanding of schizochroal eyes comes from the recent discovery of original sublensar sensory structures in the eyes of some Devonian phacopids (Figs 4a–d, 5).
It has been known for some time (Clarkson Reference Clarkson1967, Reference Clarkson, Moore and Kaesler1997; Miller & Clarkson Reference Miller and Clarkson1980; Clarkson et al. Reference Clarkson, Levi-Setti and Horvath2006) that below each lens lay a thin-walled capsule, usually with a flat base (Figs 4b, 5). Until now, nothing further was known. In the absence of further information, it was generally believed that a layer of sensory cells extended over the flat base and acted as a retina. When μ-CT-scanning became generally available, it was possible to investigate trilobite eyes in a new way (Schoenemann et al. Reference Schoenemann, Clarkson, Ahlberg, Dies Álvarez, Rábano, Gozalo Gutiérrez and García-Bellido2008b; Schoenemann & Clarkson Reference Schoenemann and Clarkson2012d, Reference Clarkson and McLeod2013). Specimens of the Middle Devonian phacopids Geesops schlotheimi (Bronn, Reference Bronn1825) (Fig. 4a), Phacops latifrons (Bronn, Reference Bronn1825) from the Ahrdorf Formation at a locality between Gees and Gerolstein, Germany and Barrandeops cf. granulops (Chatterton et al. Reference Chatterton, Fortey, Brett, Gibb and Kellar2006) from the Ma'der region, Morocco, have revealed remarkable details of original sensory structures, preserved by the ‘seeding’ of mineral films over soft parts. When the latter rotted away, the mineral films remained. Tangential scans were made at different levels below the lenses, and the upper level revealed circular rosette structures, each below a corresponding lens. These are of constant form and are regularly arranged (Figs 4c, d, 5). A second scan at a lower level showed the same kind of structures, but the rosette array was more disturbed and broken. Each rosette, arranged around a central irregularly star-shaped unit, consists of an inner circlet of six or seven wedge-shaped cells, arranged like the segments of an orange, and an outer circlet of separate black patches, each set at the outer edge of the rosette, at the junction of the cells of the inner circlet. The central star-shaped unit is interpreted as a rhabdom, the large inner cells as the sensory cells and the black patches as pigment cells. Scans made in lateral view of the Geesops eye (Fig. 4d) show several square cells with rounded terminations, and a central rod, evidently connecting with the rhabdom, around which are elongated cells, probably the sensory cells encountered in the tangential scans, or possibly pigment cells. Some other structures shown in the lateral scan remain of unknown nature.
In a specimen of Barrandeops from Morocco, the sensory cells, forming rosettes, are again visible, but preserved by a film of silica (Klug et al. Reference Klug, Schulz and De Baets2009). In another specimen of Chotecops, the sensory structures are pyritised and visible where the external lentiferous surface has been stripped off. These largely confirm what has been established by CT scanning.
Whereas, as mentioned earlier, the sensory cells were thought to be arranged as a retina, these recent investigations show clearly, if unexpectedly, that the sublensar structures much more closely resemble ommatidia, as in euarthropod apposition eyes, and particularly that of living Limulus, though much larger.
1.2.8. Eodiscid eyes
The Order Eodiscida is a group of small trilobites with only two or three thoracic segments. They are confined to the lower and middle Cambrian. Their eyes have been defined as abathochroal (Jell Reference Jell1975). This is similar to a holochroal eye, although the lenses may be somewhat separated from each other. If each lens carried its own cornea, then the abathochroal eye could be considered as a separate eye type, though this cannot be proven, and most authorities today regard the eodiscid visual system as merely another kind of holochroal eye (Clarkson et al. Reference Clarkson, Levi-Setti and Horvath2006). Eodiscid eyes are best known from phosphatised material from the lower Cambrian of China and the middle Cambrian of Australia; it is a curious fact that, despite the elegance of these eyes, many of the later eodiscids lost their visual systems altogether, for reasons which remain unknown. The eyes of many Chinese species have been studied in detail and fully illustrated elsewhere (see Zhang Reference Zhang1989; Zhang & Clarkson Reference Zhang and Clarkson1990, Reference Zhang and Clarkson1993, Reference Zhang and Clarkson2012).
1.3. Résumé and perspective
As this article shows, there is a rich record of fossil eyes that extends continuously throughout most of the Phanerozoic – from the lower Cambrian to the present day. However, the origin and evolution of vision had been a vexing problem, even for Darwin. He confessed that it was ‘absurd’ to propose that a structure as complex as the human eye could have evolved just as a result of natural selection. He found a solution, however, postulating a gradual development from “an optic nerve merely coated with pigment, and without any other mechanism” towards a “moderately high stage of perfection”, and indicated a sequence of examples of eyes with increasing complexity (Darwin Reference Darwin1859, Chapter 6). Zoologists took up his ideas directly, and produced models explaining and illustrating the pathways which led from a very simple photosensory cell, accompanied by a certain amount of pigment, to high resolving systems such as camera or compound eyes (e.g., Gehring Reference Gehring2005; Nilsson Reference Nilsson2013; Randel & Jékely Reference Randel and Jékely2016). There had been some attempts to show the evolution of complex eyes by using morphology and the fossil record alone (Young Reference Young2008). There was, however, a general consensus that the fossil record could hardly support any explanation of the evolution of eyes, because usually no soft tissues, such as nerves, sensory cells or other contributing elements of a functional eye are preserved during fossilisations. A first suggestion of how that could be challenged was given at an international conference in Nanjing (Schoenemann Reference Schoenemann2005).
Clarkson & Levi-Setti (Reference Clarkson and Levi-Setti1975) had shown, following the remarkable discoveries of Lindström in Reference Lindström1901 on the lenses of phacopid trilobite eyes, that it was indeed possible to analyse complex structures of fossilised lenses. A new perspective opened up, restoring faith in the credibility of using the fossil record to develop an understanding of at least some aspects of the evolution of visual systems through time.
The analysis of the visual systems of the lower Cambrian Chengjiang fauna, up to then regarded as ‘black spots’, was a first step forward. As noted here, the analysis of lobopodian eyes (Schoenemann & Clarkson Reference Schoenemann and Clarkson2010) as a one-lens system was the first functional analysis of a Cambrian visual system. The existence of compound eyes, probably high resolving, during the Cambrian was clear from the fossils of arthropod eyes from the Emu Bay (Lee et al. Reference Lee, Jago, Garcia-Bellido, Edgecombe, Gehling and Paterson2011; Paterson et al. Reference Paterson, García-Bellido, Lee, Brock, Jago and Edgecombe2011), and even morphological differentiation within the visual surface of complex compound eyes became apparent (Zhao et al. Reference Zhao, Bottjer, Hu, Yin and Zhu2013). By adapting the physical tools (Horridge Reference Horridge1977, Reference Horridge1978; Snyder Reference Snyder1977, Reference Snyder and Autrum1979; Land Reference Land and Autrum1981) developed to describe the performances of extinct systems, it was possible to develop further differentiated insights into vision in the Cambrian. It was possible in such a way to assign the organisms of the Chengjiang fauna to their original habitats, by estimating their eye parameter and sensitivity (Schoenemann & Clarkson Reference Schoenemann and Clarkson2010, Reference Schoenemann and Clarkson2011, Reference Schoenemann and Clarkson2012a, Reference Schoenemann and Clarksonb, Reference Schoenemann and Clarksonc); whilst the results were in good accordance with the palaeoecological interpretations of the Chengjiang biota of Chen & Zhou (Reference Chen and Zhou1997). As shown here, the eye parameter had been applied in trilobites before (Fordyce & Cronin Reference Fordyce and Cronin1989, Reference Fordyce and Cronin1993; McCormick & Fortey Reference McCormick and Fortey1998). Its successful application, even to Cambrian arthropods, seems to have triggered a number of analyses of fossil compound eye systems in other arthropods of Cambrian time (Zhao et al. Reference Zhao, Bottjer, Hu, Yin and Zhu2013), trilobites (Schoenemann & Clarkson Reference Schoenemann and Clarkson2015; Tanaka et al. Reference Tanaka, Schoenemann, El Hariri, Ono, Clarkson and Maeda2015), crustaceans (Schoenemann & Clarkson Reference Schoenemann and Clarkson2012a, Reference Schoenemann and Clarksonb, Reference Schoenemann and Clarksonc; Rust et al. Reference Rust, Bergmann, Bartels, Schoenemann, Sedlmeier and Kühl2016; Vannier et al. Reference Vannier, Schoenemann, Gillot, Charbonnier and Clarkson2016) and eurypterids (Anderson et al. Reference Anderson, McCoy, McNamara and Briggs2014; Poschmann et al. Reference Poschmann, Schoenemann and McCoy2016). It may be concluded that this is indeed an effective way of investigating the structural parameters in the eyes of fossils. From early Cambrian times, we have simple compound eyes with spherical dioptric units and complex hexagonal patterns of facets, and we find one-lens systems such as in the lobopodians, or the median eyes of euarthropods, as discussed above.
The disadvantage for any model about the evolution of eyes, especially when considering those of extinct organisms, and the incomplete nature of the fossil record, is that one cannot be overly confident about what actually happened during the course of time; thus the conclusions remain purely hypothetical. A recent breakthrough came when the sensory structures of phacopid compound eyes were first made visible by µ-CT and synchrotron analyses (Schoenemann et al. Reference Schoenemann, Clarkson, Ahlberg, Dies Álvarez, Rábano, Gozalo Gutiérrez and García-Bellido2008b, Reference Schoenemann, Clarkson, Ahlberg and Dies-Alvarez2010; Schoenemann & Clarkson Reference Schoenemann and Clarkson2012d, Reference Clarkson and McLeod2013). Even if these structures are still not completely understood, this work opened the way for studying preserved soft tissues of eye structures to understand their function, internal complexity and evolutionary significance. In 2014, Tanaka et al. were able to show the rods and cones inside a fish retina, indicating that this fish possessed colour vision. Other recent discoveries by Strausfeld and co-workers showed that in at least some Chengjiang fossils, the delicate architecture of arthropod brains can be preserved, including the optical lobes (Ma et al. Reference Ma, Hou, Edgecombe and Strausfeld2012; Cong et al. Reference Cong, Ma, Hou, Edgecombe and Strausfeld2014; Strausfeld et al. Reference Strausfeld, Ma, Edgecombe, Fortey, Land, Liu, Cong and Hou2015). This gave the potential for further progress into analysing the relationships amongst Cambrian arthropods and modern organisms. The future holds the potential for inclusion of valuable data from the fossil record – especially that derived from modern technologies – in developing new perspectives on the evolution of vision. This, more than any models referring to Recent organisms alone, will allow us to better understand how eyes originated and evolved, and even solve some long-standing evolutionary conundrums (e.g., Clements et al. Reference Clements, Dolocan, Martin, Purnell, Vinther and Gabbott2016), because we have the witnesses posted along the long axis of evolutionary time.