Introduction
The Copernican principle posits that there is nothing special about us in the Universe, ergo, ETI must exist. The Copernican anthropic principle for predicting the future (Gott, Reference Gott1993) may be cast into Bayesian form (Griffiths and Tenenbaum, Reference Griffiths and Tenenbaum2000). The time at which an observer detects a phenomenon (such as the detection of extraterrestrial intelligence) is random. The time elapsed since the start of a random phenomenon t past (development of technological intelligence, say 10 000 years) is related to the total duration of the phenomenon ttotal (lifetime of technological intelligence) through the r ratio: 0 < r = (t past/t total) < 1. Randomness proposes that r < 0.5 with the probability that the future is longer than the past, p(t past<t future) = 0.5. For 95% confidence, 0.05<r < 0.95 and t future = t total − t past, then (1/39)t past < t future < 39t past. Given the randomness of events, the prior is given by p(t total) ∝ (1/t total) and the likelihood is given by p(t past|t total) = (1/t total). The probability of observing n exemplars of ETI detections is given by (1/t total)n. The posterior time is thus given by: t = t past + (t past/n). Hence, we should observe ETI contact imminently as the number of ETI grows as n → ∞. This assumption motivates SETI. Current SETI methods are based on searching the sky for extraterrestrial radio signals. Despite 60 years of searching for such signals including in the ‘waterhole’ bounded by the H-line at 1420 MHz and the OH line at 1670 MHz, no reliable ETI signals have been detected (though I would contend that this is not the most efficacious mode of search). Using Bayesian analysis, the current non-detection of radio signals means that there can be no more than ~100 undiscovered Earth-crossing Arecibo-power radio signals originating within 1000 light years with a 90% probability (Grimaldi and Marcy, Reference Grimaldi and Marcy2018). Ruling out such radio signals entirely requires non-detection in an all-sky survey out to 40 light years. Although fast (millisecond) powerful radio bursts (primarily from FRB121102, a dwarf galaxy 3 × 109 ly from Earth) may be microwave sources used for propelling extraterrestrial spacecraft, they are more plausibly postulated to be the result of magnetars that also produce gamma ray bursts and X-ray flares due to the release of enormous amounts of magnetic energy (Chatterjee, Reference Chatterjee2021). The magnetar hypothesis for radio bursts is disputed with recycled pulsars offering an alternative candidate source (Ravi, Reference Ravi2022). The recent advent of the Square Kilometre Array (SKA), optical SETI at the Lick and Keck observatories, and Breakthrough Listen offer enhanced search options but the prospects for successful detection of extraterrestrial radio signals are diminishing over time. A recent (2019) candidate Breakthrough Listen detection from Proxima Centauri by the Parkes radio telescope in Australia was declared a terrestrial false alarm generated by electronics (Witze, Reference Witze2021). Furthermore, if ETI are adopting spread spectrum methods for targeted communication, their signals are broadened across high bandwidth and exhibit high noise-like properties giving them reliable interstellar transmission with security (Messerschmitt, Reference Messerschmitt2012). This will make them impossible to detect unless the pseudorandom code is deliberately easily cracked, e.g. digits of π, e, etc.
Active SETI proposes transmitting messages to prospective ETI into deep space from Earth. The four potential ‘dangers’ of this strategy are weak arguments (Musso, Reference Musso2012): (i) the cultural shock of the discovery of ETI is unlikely to occur except in those with delicate religious sensibilities; (ii) any malicious viral signal cannot infect terrestrial systems without detailed knowledge of the target's design (such as stuxnet); (iii) alien technological superiority potentially imposes competitive exclusion which requires direct physical interaction, e.g. extinction of Neanderthals on exposure to invading Cro-Magnons; (iv) alien invasion is no more likely to result from active SETI than from our radio leakage into the local interstellar environment. Furthermore, despite the (low) security risk, the probability of success is extremely low ~10−12 (Ashworth, Reference Ashworth2016).
Search for Technosignatures
Interstellar archaeology involves the search for technological signatures at astronomical scales (Carrigan, Reference Carrigan2012) such as Dyson spheres and other evidence of stellar and galactic engineering. We are in urgent need to transition towards a Kardashev type I civilization (exploiting 2 × 1016 W of solar energy intercepted by the Earth). If we develop solar power satellites as a source of clean energy (Glaser, Reference Glaser1992), our current needs of ~1013 W require a geostationary ring ~1 km in width (Ellery, Reference Ellery2016b). The waste heat generated will radiate to deep space at thermal infrared wavelengths. Although planetary rings will be difficult to detect, as our energy needs grow, so will the width of the solar power satellite ring potentially enveloping the planet – the infrared signature could become increasingly detectable. The O'Neill colony may constitute a transitioning step from a Kardashev type I to type II civilization as industry migrates away from the Earth's biosphere (O'Neill, Reference O'Neill1974). The O'Neill colony comprises a pair of coupled cylinders 6.4 km diameter by 26 km length with controllable day/night cycles using mirrors, implementing artificial gravity through two-minute period rotation and fashioned with internal environments including agriculture, housing for 200 000 people, savannah, lakes, etc. Multiple such colonies could form a ring at 1 AU around the Sun. The Dyson shell may be regarded as an evolution of this for a Kardashev type II civilization collecting and trapping its solar energy and re-radiating waste heat at thermal infrared wavelengths centred near 10 μm (Dyson, Reference Dyson1960) (though no evidence of Dyson spheres has been gleaned from infrared satellite searches). By harvesting the energy of a star, this would constitute a Kardashev type II civilization (exploiting solar energy of 4 × 1026 W). Other forms of stellar engineering might be observable such as increasing stellar longevity – blue stragglers are natural rather than artificial phenomena resulting from increased mixing due to stellar collisions in globular clusters thereby extending their main sequence lifetimes. An alternative to extending main sequence lifetime is to bleed mass from the star by projecting a collimated electromagnetic laser/maser beam into it (star lifting), e.g. one candidate is the F3 pre-subgiant star KIC8462852 due to its anomalous light curve (Matloff, Reference Matloff2017). This is speculative at this stage. Other signs such as ETI indulging in asteroid mining in its stellar system would not be readily detectable from Earth via the properties or characteristics of circumstellar dusty discs (Forgan and Elvis, Reference Forgan and Elvis2011). For large-scale galactic engineering, there is no evidence of significant clusters of Dyson spheres.
Rather than SETI searches which rely on an array of assumptions, searching for interstellar (Bracewell) probes is an alternative approach (Bracewell, Reference Bracewell1960). The transmission of information across interstellar distances may be conveyed through energy (electromagnetic radiation) or matter (interstellar probes) but only the latter offers military security to the sending civilization (Freitas, Reference Freitas1980a). Colonization dynamics may be modelled as a percolation problem in which colonizing (driven by non-sustainability limits) civilizations will form clusters linked into fractal structures through surrounding non-colonizing (imposed by sociological constraints) civilization regions (Landis, Reference Landis1998). The interstellar bandwidth theory suggests that there is a limit to physical migration between star systems (Wiley, Reference Wiley2011). Such models do not apply to self-replicating probes since they exhibit exploration rather than colonization rationales and strategies which is guaranteed to fill the Galaxy exhaustively and so are better modelled as exhaustive approaches (Jones, Reference Jones1978). The average age of stars in the Galactic habitable zone is 1.8 Gy older than our Sun (Lineweaver et al., Reference Lineweaver, Fenner and Gibson2004). This suggests a stark motive for exploration – necessity due to the evolution of the home star to late main-sequence phases. It takes only one such intelligent species to embark on a programme of interstellar exploration. It has been suggested that an interstellar network of communication nodes set up by interstellar robotic probes does not suffer most of the ad hoc assumptions and limitations of targeted radio searches by ETI (Gertz, Reference Gertz2021). An effective medium to implement such a strategy would be self-replicating probes. Self-replicating probes are superior to long-lived single-shot (Bracewell) probes for any enduring interstellar exploration programme (Valdes and Freitas, Reference Valdes and Freitas1980). Self-replicating probes are the minimum cost approach to the exponential exploration of our Galaxy and beyond.
The only candidate artificial extraterrestrial visitor we have had in the Solar System was ‘Oumuamua’ in 2019. ‘Oumuamua’ was an extrasolar asteroid that travelled at 27 km s−1 indicating a hyperbolic escape trajectory. It was estimated from a light curve variation of 10:1 to be a triaxally tumbling 400 m × 40 m cigar-shaped object with a high albedo >20% and period of <8 h (Glester, Reference Glester2019). In a hyperbolic orbit with an ellipticity of 1.2, it passed within 25 Mkm of Earth having originated from near Vega in the Lyra constellation 25 light years away before slowly accelerating out of the solar system to the constellation of Pegasus. The acceleration may be due to outgassing through solar heating but no evidence of gas or dust was observed. The postulation that ‘Oumuamua comprises solid hydrogen or nitrogen is unlikely as such comets have never been observed otherwise. A flat solar sail with tip-vanes for manoeuvrability has been proposed as consistent with the observations. A variation on this theme is solar thermal propulsion which offers higher efficiency by using thermal energy to heat and accelerate hydrogen propellant (Sheerin and Loebb, Reference Sheerin and Loebb2021). An interceptor could potentially be launched using a Jupiter gravitational assist to intercept ‘Oumuamua's trajectory (Lunan and Coppinger, Reference Lunan and Coppinger2021). On average, two stars pass within 1 light year of our Sun every 500,000 years rising to 200 within 10 light years. If a fraction f of N stars launched a lurker probe over the last 2.5 billion years, N/f~500,000 probes should have encountered near-Earth space (Benford, Reference Benford2021). The most accepted theory is that ‘Oumuamua is an interstellar asteroid resembling an organic-rich comet or a D-type asteroid (Meech et al., Reference Meech, Weryk, Micheli, Kleyna, Hainaut, Jedicke, Wainscroat, Chembers, Keane, Pertric, Denneau, Magnier, Berger, Huber, Flewelling, Waters, Schunova-Lilly and Chastel2017). Interstellar spacecraft themselves might be detected from Earth by their high-speed signatures such as γ-ray or X-ray emissions (Zubrin, Reference Zubrin1995) and extreme Doppler-shifted reflected light from solar sails (Garcia-Escartin and Chamorro-Posada, Reference Garcia-Escartin and Chamorro-Posada2013). Self-replicating probes may be microscale in size ~0.1 μm, replicating in HII regions but may be detectable with a characteristically exponentially increasing luminosity in the infrared spectrum (Osmanov, Reference Osmanov2020). There is no evidence of such.
There are several candidate locations for extraterrestrial artefacts (lurking spacecraft, artificial civil engineering structures, underground repositories, etc) as evidence of extraterrestrial exploration using interstellar probes (Freitas, Reference Freitas1983a, Reference Freitas1983b): (i) Earth as a tectonically active body does not offer a geologically stable location for artifacts – even evidence of a full industrial civilization and its climatic effects deep into Earth's geological history (Silurian hypothesis) would have similar multivariate geological signatures to mass extinction events (Schmidt and Frank, Reference Schmidt and Frank2017) rendering them difficult to detect; (ii) the Moon (or moons) acts as a stabilizing influence on the primary body and has been tectonically inactive for 2 billion years though its surface undergoes impact gardening over 106 y – the Moon appears to act as a collector of artefacts (Arkhipov, Reference Arkhipov1995, Reference Arkhipov1998); (iii) main asteroid belt or Near-Earth asteroids (but the latter have short residence times ~108 y) for infrared emissions due to mining activity during self-maintenance and self-repair (Oort cloud and Kuiper belt objects are too far from the sun to exploit it as an abundant energy source though the latter have been proposed as targets (Matloff and Martin, Reference Matloff and Martin2005)) – there are around 10 000 C-type asteroid candidates that would require a resolution of 10 cm/pixel to detect human-scale artifacts (Kecskes, Reference Kecskes and Badescu2013); (iv) stable Lagrangian L4 and L5 points around bodies of interest such as Sun-Earth and Earth-Moon – halo orbits around the Earth-Moon L4 and L5 points have been searched optically to 14th magnitude without success (Freitas and Valdes, Reference Freitas and Valdes1980b); (v) the focus of the Sun's gravitational lens outward from 550 AU (Maccone, Reference Maccone2008); (vi) emissions from a decelerating extraterrestrial spacecraft (even if of diminutive size ~1–10 m or employing camouflage or stealth technology (Haqq-Misra and Kopparapu, Reference Haqq-Misra and Kopparapu2012) approaching the solar system off-the-ecliptic to minimize particle flux impingement, e.g. gamma rays from antimatter drives; (vii) debris from astronomical-scale mining may be detectable as thermal signatures of dust in debris discs around evolved stars but would be indistinguishable from natural processes (Forgan and Elvis, Reference Forgan and Elvis2011); (viii) radio or laser communication transmissions from extraterrestrial civilizations (currently, the main strategy of SETI programmes). There is no evidence of such.
However, the solar system has not been systematically mapped with high resolution sufficiently to exclude undetected probes (Freitas, Reference Freitas1983a, Reference Freitas1983b). We propose that searching the asteroids for signs of past mining activities would be a reasonable strategy to search for signs of earlier visitations of self-replicating probes. There are no signs of dormant Bracewell probes in the asteroid belt (Papagiannis, Reference Papagiannis1978) nor at the Sun-Earth or Earth-Moon Lagrangian points from optical searches (Freitas and Valdes, Reference Freitas and Valdes1980b). There are no large interstellar spacecraft, be they gossamer or worldship, in evidence in the Solar System. Although this doesn't exclude diminutive spacecraft (Haqq-Misra and Kopparapu, Reference Haqq-Misra and Kopparapu2012), the self-replicating machine requires a degree of stature that is not readily concealed, particularly if adopting laser-propelled lightsails or variants thereof. Other possibilities include indirect evidence of prior visitation. As our own asteroid belt represents a source of material resources, the residue of resource extraction might be detectable. This represents a more subtle trace of extraterrestrial visitation. The Moon has been mapped to a resolution of 0.5 m by the Lunar Reconnaissance Orbiter but no extraterrestrial artefacts either intentional (such as a buried beacon akin to radio beacons implied by SETI) or unintentional (such as from mining/construction activities or nuclear waste) have been found (though lava tubes represent an unexplored possibility) (Davies and Wagner, Reference Davies and Wagner2013).
Self-replicating probes in SETI
One of the first major comprehensive studies on self-replicating machines – the NASA/ASEE 1980 summer study on advanced automation for space missions (Freitas and Gilbreath, Reference Freitas and Gilbreath1980a; Freitas et al., Reference Freitas, Healy and Long1982) – outlined a concept for a self-replicating lunar factory to robotically industrialize the Moon using local lunar resources. A modest but more recent experimental setup demonstrated self-assembly aspects of a self-replicating lunar rover (Chirikjian, Reference Chirikjian2002). The Moon of course offers a potentially valuable location for both self-replication and/or nanotechnology experimentation without danger to Earth. The first self-replicating probe concept was proposed as the payload for a suitably scaled Daedalus starship design with a 0th stage to permit deceleration at the target star system rather than a flyby (Freitas, Reference Freitas1980a, Reference Freitas1980b). Its dominant mass is nuclear fusion propellant D/3He mined from Jupiter's atmosphere. The payload comprised a 450-tonne robotic factory that grows itself to full capacity on landing. It comprised: (i) power systems, (ii) computer controllers, (iii) aerostats, (iv) tankers, (v) mining excavators, (vi) haulers, (vii) chemical processors, (viii) material processors, (ix) fabrication laboratory, (x) assembly robots, (xi) warehousing, (xii) maintenance and repair wardens and (xiii) verification testers. Some of these tasks can be merged using modern technology such as solid-state electrochemical processing (merging aspects of chemical and material processing), 3D printing (merging aspects of fabrication and assembly), reconfigurable manipulators (merging aspects of assembly and maintenance/repair), just-in-time manufacturing (eliminating warehousing), etc. Aerostats and tankers can be eliminated if adopting propellantless propulsion.
Searching for self-replicating probes might be a more rational strategy than searching for electromagnetic signals. One medium of investigation and communication with extraterrestrial intelligence is locally through the internet which requires interstellar probes – this is the rationale behind the ‘Invitation to ETI’ (http://ac.ieti.org/) (Tough, Reference Tough1998). The idea of the self-replicating probe (we shall avoid the term ‘von Neumann probe’ due to its propensity for confusion with the more well-established term ‘von Neumann architecture’ and the generalized and more nebulous term ‘von Neumann machine’ used to refer to both despite being entirely different concepts) to explore the Galaxy was invoked by Frank Tipler as an argument against the existence of ETI (Tipler, Reference Tipler1980). Tipler was enunciating an earlier argument by Michael Hart (Hart, Reference Hart1975). Hart began with his Fact A that ETI do not exist on Earth now. There are only four explanations for this: (i) interstellar travel is impossible – this is dispensed with later; (ii) ETI choose not to explore but sociological explanations cannot apply to all civilizations at all times, e.g. zoo hypothesis (Ball, Reference Ball1973); (iii) ETI haven't arrived but this postulates that the Universe is in special state transition between stable states; (iv) ETI have visited in the past (von Daniken hypothesis) for which there is no evidence. Despite the power of the Hart-Tipler arguments scientifically, their personal viewpoints were considered controversial – in the case of Tipler, it is his Christian Teilhard de Jardin-type theology that rankles; in the case of Hart, it is his unsavoury white supremacist leanings. This has (wrongly) tainted their scientific arguments. In particular, the opposing argument espoused by the admirable Carl Sagan, illuminatus extraordinaire, has held sway primarily because of his benign influence over several generations of scientists (including myself) who will brook no ill towards him. A review of the Tipler-Sagan debate is given in (Ellery et al., Reference Ellery, Tough and Darling2003).
The essence of the Hart-Tipler argument is that a self-replicating spacecraft would be sent to any neighbouring star system and use the raw materials available therein to manufacture copies of itself to be sent thenceforth to other star systems ad infinitum. Local stellar density of stars is ~0.004 ly−3 but only ~20% of planets are expected to reside in the habitable zone of stars. This reduces stellar density to ~0.0008 ly−3 for astrobiological targets but self-replicating probes may be employed in an exhaustive search to all stars for resources regardless of scientific or philosophical interest – it is access to physical resources within minimum transit that is the driver of a self-replicating system. The self-replicating spacecraft would arrive at its destination star system and exploit raw materials from asteroids and moons to construct copies of itself. Around 60% of Sun-like stars exhibit evidence of terrestrial-type planets. We expect the asteroids and moons of other star systems to have similar constitutions to those of our own solar system given the ubiquity of the laws of chemistry ceteris paribus. Planets are formed through the accretion of a disc of gas and dust forming a well-defined condensation sequence though subsequent planetary migrations may distort this sequence. Most common rock-forming minerals are silicates formed from magma which cools – different minerals concentrate and crystallize at different temperatures, e.g. feldspar is an early precipitating mineral. As each mineral crystallizes, the magma composition changes. Bowen's reaction series predicts that certain minerals form in association with each other while other minerals are never associated with each other. This is because of the conditions in the cooling magma. Similarly, the Goldich dissolution series predicts the rate of weathering based on the stability of minerals on Earth. Minerals that form at higher temperature/pressure are less stable on the surface than those formed at lower temperature/pressure. Both series predict that mafic silicates weather more than quartz.
We expect asteroid belts to be a common feature of stellar systems as indicated by thermal emissions from dusty discs around 10–30% of main-sequence stars. Asteroid belts in the form of accretion discs occur around 18% of stars aged 3–30 My old dropping to 12% at age 30–300 My and 2% at 0.3–3 Gy old but significant asteroid remnants are expected to remain. There may be variations to this around Population I stars which are metal-deficient but there also exist star systems with super-Earths with masses up to 10 ME indicating enhanced metallicity. Asteroid belts are expected to form at the snow line at 170 K of a stellar system due to the formation of giant planets just outside the snow line (Martin and Livio, Reference Martin and Livio2013). The asteroid belt location is weakly dependent on stellar mass as R snow ≈ 2.7(M star/M Sun)1/3 AU. Planetary migrations can deplete the asteroid belt – our asteroid belt originally contained an Earth mass of asteroids that was subsequently disrupted to 0.1% of its original mass by Jupiter's 0.2–0.3 AU migrations even though they occurred twice as far as the snow line. Our own asteroid belt would be undetectable indicating that these measured thermal emissions are from stellar systems in which the giant planets formed far from the snowline near the end of the protoplanetary disc lifetime preventing planetary migration and so asteroidal dispersion. Because stars and their rocky planets accrete from the same nebula, their respective compositions as measured by their Fe/(Mg + Si) mass fractions is correlated (Adibekyan et al., Reference Adibekyan, Dorn, Sousa, Santos, Bitsch, Israelian, Mordasini, Barros, Mena, Demangeon, Faria, Figueira, Hakobyan, Oshagh, Soares, Kunitomo, Takeda, Jofre, Petrucci and Martioli2021) so rocky asteroidal material composition may be inferred remotely.
Asteroids offer the gamut of raw materials for feeding the self-replicating machine – metals, ceramics, volatiles (presumed to be similar in nature as lunar volatiles), reagents and feedstock for plastics. We can assume, with minor variations, solar abundances may be typical of population II stars in general (Table 1) (Ringwood, Reference Ringwood1966).
Table 1. Solar system elemental abundances
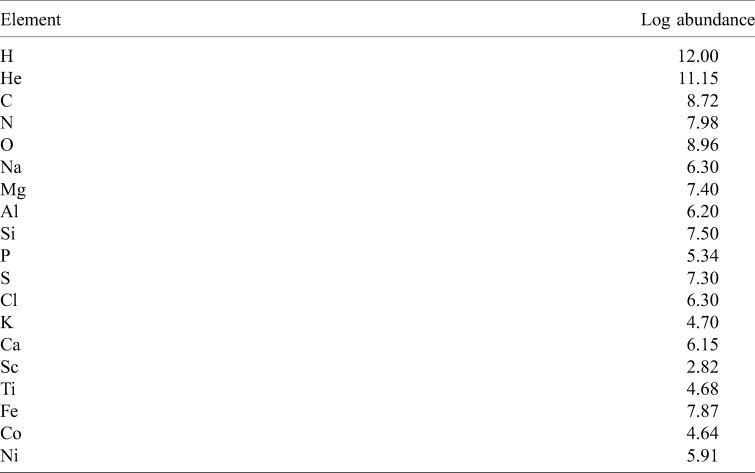
We select A-type asteroids as representative of both M-type and S-type in that they comprise a median species with 50% olivine Mg2SiO4 and pyroxene (dominantly enstatite MgSiO3 which is also representative of E-type asteroids) silicate grains embedded in a 50% matrix of Fe-Ni-Co metal alloy with some FeS inclusions. Carbonaceous material from C-type asteroids would offer a critical source of salts, carbon and a hydrogen source from water for any number of applications. One of the chief challenges in asteroid mining will be in conducting mining and processing operations under the microgravity environment imposed by asteroids. One possibility that has yet to be fully explored is the biomimetic drill concept based on the wood-wasp (Sirex noctilia) ovipositor mechanism (Vincent and King, Reference Vincent and King1996; Gao et al., Reference Gao, Ellery, Vincent, Eckersley and Jaddou2007). It employs two longitudinal sections that penetrate through a dual-reciprocating mechanism – the two segments of the drill stem alternate between anchorage and penetration, the anchored segment providing traction for the percussive digging segment. The anchoring mechanism potentially eliminates the weight-on-bit force required for drilling but there is a requirement for initial anchorage that remains – impact penetration by harpoon is one possibility.
Self-replicating probes are an example of TRIZ (Teorija Reshenija Izobretatel'skih Zadach), a theory of inventive problem solving that matches biomimetic solutions to technological problems (Vincent et al., Reference Vincent, Bogatyreva, Bogatyrev, Bowyer and Pahl2006). The self-replicating machine may be deemed an inevitable result of industrial production: the Griliches production function defines productive output Y given productive inputs X of capital and labour and scientific knowledge K of process/product innovations by: logY = βlogX + γlogK. In a robotic self-replicating machine, labour is eliminated through autonomous capability and capital investment in the machines of production equates to (low-cost) raw material only (X ≈ 0) as machines of production (capital) now constitute the productive output Y, i.e. logY ≈ γlogK. The production function becomes dependent only on scientific knowledge. The technological problem is to economically explore our interstellar neighbourhood with minimum capital investment – the biomimetic solution is to exploit the exponential growth in productive capacity rendered by self-replicating systems. As self-replicating machines permeates the Galaxy, the population of self-replicating machines grows thus:

where r = number of offspring per generation, m = number of generations. The entire Galaxy could be colonized within 24 generations (yielding a population of 424 billion) assuming two offspring per generation, i.e. the maximum number of offspring is constructed by the first generation of probes at 48. If the number of offspring per generation is increased to three, this reduces Galactic colonization time to 20 generations with a maximum of 80 offspring for the first generation, i.e. beyond two offspring per probe, there is little generational performance gain with more offspring per generation. We suggest that replication time is short – a span over only a few years at most so colonization time is dominated by the transit time between stars which we take to be 100 years at 0.1 c initially but slowing as the population occupies local space. Time will then be determined by the transit time across the 100 000 light year diameter Galaxy, i.e. ~1 million years. Given this short timespan compared with the age of the Galaxy, our Galaxy should be swarming with self-replicating probes yet there is no evidence of them in our solar system. Indeed, it only requires a civilization to exist long enough to send out such probes as they would thenceforth continue to propagate through the Galaxy even if the sending civilization were no more. And of course, it requires only one ETI to do this. Thus, the Fermi paradox or Great Silence (Brin Reference Brin1983) emerges: if extraterrestrials exist, where are they? There exist a host of ad hoc explanations (Webb, Reference Webb2002), some bordering on solipsism such as the virtual planetarium (Baxter, Reference Baxter2001) but all sociological arguments are flawed because they must apply universally (Hart, Reference Hart1975). The simplest hypothesis to draw from this (lack of) evidence is that ETI do not exist – a direct application of the Occam's razor principle. See Ellery (Reference Ellery2003) for a justification of Occam's razor principle from a computational complexity theory perspective. Although primarily applied to our own Galaxy, the Fermi paradox may be extended to 2.5 million galaxies assuming intergalactic transits at 0.5 c by a relativistic rocket over the last 2 billion years (median age of terrestrial planets) (Armstrong and Sandberg, Reference Armstrong and Sandberg2013). The Great Filter notion postulates that there is a bottleneck in the Drake equation (N = Rf sf pn ef lf if tL where R = rate of star formation in the Galaxy, f s = fraction of stars with habitable zones, f p = fraction of stars with planets, n e = Earth-like fraction of planets, f l = fraction planets that give rise to life, f i = fraction of species that spawn intelligence, f t = fraction of intelligence that spawn technological civilization, L = lifetime of technological civilization) (Hanson, Reference Hanson1998) such as the lifetime L of an extant civilization being short.
All existential risks are Great Filters that threaten wholesale human (or other intelligent species) survival and have no precedent in human history because they result primarily from technological advances (Bostrom, Reference Bostrom2002): (a) sudden disasters by (i) runaway global greenhouse warming, (ii) all-out nuclear holocaust, (iii) global extinction by asteroid or comet impact, (iv) pandemic by natural or genetically engineered doomsday virus, (v) deliberate or accidental misuse of molecular nanotechnology (grey goo scenario), (vi) inadvertent destruction by high energy physics experiment, (vii) universe simulation halted and (viii) extermination by malignant superintelligence; (b) prevention of progress by (i) resource depletion or ecological destruction without replenishment, (ii) social stagnation preventing technological progress through oppression or genetic suppression and (iii) technological ceilings imposed by physical limits; (c) narrow progress by (i) take-over by mind-uploaded elite, (ii) take-over by flawed superintelligence and (iii) global regression to totalitarian elite; (d) progress in undesirable directions by (i) evolutionary de-selection of human core values and (ii) extermination by extraterrestrial encounter. In all cases, there are of course unforeseen factors. These filters cannot explain the lack of self-replicating probes as they propagate independently of the survival time of the sending civilization. The only explanation left consistent with the astrobiology paradigm that microbial life is a universal physicochemical process is that multicellular species (and so, technologically intelligent species) rarely evolve from biological life (the rare Earth hypothesis) (Ward and Brownlee, Reference Ward and Brownlee2003). The rationale is based on several factors: (i) correct planetary mass in the habitable zone for plate tectonics; (ii) Jupiter-sized gas giant in the outer solar system; (iii) large orbiting moon to stabilize homeworld axial tilt; (iv) habitable zone of a G2 star within the larger habitable zone of the Galaxy for metallicity. For example, the Jovian system ensures that catastrophic impacts yielding major climatic changes occur every ~106 y to stimulate evolutionary novelty. Technological intelligence provides a means to mitigate against such impacts (Ellery, Reference Ellery2020a) though long-period comets from the Oort cloud present a greater challenge than asteroids as they are more difficult to detect prior to the formation of a comet tail through volatile evaporation near Jupiter's orbit.
We must now consider how we might implement a self-replicating interstellar probe. Full autonomy is essential for interstellar flight and extrasolar planet investigation in the search for evidence of life and intelligence (Ellery, Reference Ellery2010). A high degree of onboard intelligence is required to perform tasks from sophisticated image processing, reasoning, planning and decision-making as well as more creative cognition such as learning and hypothesis generation. There is no scientific or technological reason why highly capable artificial intelligence cannot be created. Any interstellar probe, especially a self-replicating one, requires a high degree of autonomy to adapt to different stellar system environments. It requires scientific instruments to search for specific features which are limited by technological sophistication. Any basic scientific suite must include telescopy in the X-ray-ultraviolet-visible-infrared-radio spectrum for long-range reconnaissance. Scientific autonomy requires an autonomous search for signatures and their interpretation – this is an under-developed field (Ellery, Reference Ellery2018b). Adaptability to environmental exigencies implies learning and/or evolutionary capacity. One significant problem with neural learning is that it learns polynomial-like input-output patterns from large datasets rather than underlying algorithmic principles. This may be exemplified by the transfer learning problem of adapting robot manipulator control on Earth to the zero-gravity environment in space. The control algorithms have the same form with minor parameter adjustments (Ellery, Reference Ellery2004) but neural learning of the latter requires complete re-training from new input-output data sets (Ellery, Reference Ellery2020d). Even enhancement of the backpropagation algorithm with Kalman filtering which enriches the learning process does not address this problem. Yet explicit control algorithms offer insights into the underlying dynamics – this suggests that fruitful research should be directed towards hybridizing symbolic with neural techniques (Ellery, Reference Ellery and Ngo2015). It is crucial that motor capabilities are mastered as the evolutionary history of animal neural structures has been stimulated by advances in motor capability – central pattern generators for fish undulation evolved for limb stepping in land animals; central pattern generators for legged locomotion evolved into decoupled controllers for arms and hands in primates. Autonomic computing currently under development is the hardware equivalent characterized by the following properties (Ganek and Corbi, Reference Ganek and Corbi2003): (i) self-configuring, (ii) self-healing, (iii) self-optimizing, and (iv) self-protecting, i.e. robust.
To exceed its own programming and self-replicate uncontrollably, the self–replicator must be able to inspect itself, re-design itself, and design its own programs. Both program and hardware design may be implementable through genetic algorithms and self-inspection may be possible using extensive sensor suites with hidden Markov models. However, it is relatively straightforward to restrict these capabilities. Hybrid neural-symbolic systems offer a promising approach which may be implemented in read-only format to prevent learning, and so, uncontrolled behaviour (Ellery, Reference Ellery and Ngo2015, Reference Ellery2019a). Hence, the self-replicating probe cannot deviate from its programmed instructions. Genetic invariance must also be implemented. Mutation is most likely to yield abject failure due to brittleness rather than enhanced performance. Furthermore, M-of-N redundancy through multiple program copies and error detection and correction codes (EDAC) may be employed in a self-replicating machine to impose a Hayflick limit on the number of replications permitted. Additionally, evolutionary change may be effectively halted through the same approach of redundancy and EDAC (Ellery and Eiben, Reference Ellery and Eiben2019a). Any kind of channel code adds structured redundant bits to increase the fidelity of information transmission at the cost of higher bandwidth requirements. The SNR can be related to bit error per noise E b/N 0 by:

where R = data rate, B = bandwidth. Above the theoretical Shannon coding limit, there is a code that can communicate with zero error (Costello and Forney, Reference Costello and Forney2007):

For example, the Golay code is a ‘perfect’ three-error block code that works only for (23,12) for d = 7 and (24,12) for d = 8 – it is based on a remarkable number theoretic relation ${{2^{23}} \over {\left({\matrix{ {23} \cr 0 \cr } } \right) + \left({\matrix{ {23} \cr 1 \cr } } \right) + \left({\matrix{ {23} \cr 2 \cr } } \right) + \left({\matrix{ {23} \cr 3 \cr } } \right)}} = ( 2^{23}/2^{11}) = 2^{12}$ where $\left({\matrix{ n \cr k \cr } } \right) = ( {n!/( {n-k} ) !k!} )$
= binomial coefficients. It would be curious indeed if evolution had discovered the tri-error Golay code for the terrestrial tri-base genetic code but it and its variants may be employed in self-replicating machines to prevent evolutionary change over the 24 generations required. These codes may be interleaved and embedded to an arbitrary degree (at the cost of memory storage). EDAC prevents grey-goo scenarios of runaway replication (though this is usually attributed to nanotechnological assemblers rather than clanking replicators) (Joy, Reference Joy2000). Nanotechnology concepts of self-replicating machines were based on the nano-assembler, a conceptual example of which is a 0.1 μm molecular manipulator of carbon (nanotube-graphene-fullerene) in a Stewart platform configuration for precise positional control and tip chemistry for manipulating molecules similar to the ribosome (Merkle, Reference Merkle, Welland and Gimzewski1994). The Smalley-Drexler debate introduced the fat and sticky fingers problem to nanotechnology that molecular assemblers cannot manipulate atoms because they are themselves constructed from multiple atoms and cannot be positioned with arbitrary position and atoms stick together due to van der Waal forces (Tourney, Reference Tourney2018) – biology overcomes this through molecular recognition and enzymes in a solvent that facilitates self-assembly. However, a molecular assembler has been demonstrated that reversibly rotary switches between two activating sites of opposing chirality controlled by the addition or deletion of a proton (Kassem et al., Reference Kassem, Lee, Leigh, Marcos, Palmer and Pisano2017; Kelly and Snapper, Reference Kelly and Snapper2017). This suggests that nanotechnology-scale self-replication is feasible but we do not consider it here.
An important facet is that self-maintenance and self-repair capability are implicit in self-replication capability which itself affords robust exponential population growth. Failure modes such as transit, foothold and production failures (Fow, Reference Fow2021), are unlikely prevent the spread of self-replicating probes. It has been suggested that self-replicating probes might mutate into a predator-prey ecology (Forgan, Reference Forgan2019). A reaction-diffusion version of the Lotka-Volterra model of such an ecology through the Galactic Habitable Zone exhibited oscillatory populations followed by equilibrium with the spread of high prey populations so the Fermi paradox remains. Nevertheless, the significant evolution of a predator-prey ecology is unlikely given the small number of generations required to populate the Galaxy. Due to finite genetic fidelity during self-replication, it has been asserted that such an evolutionary process will inevitably yield a runaway error catastrophe due to the accumulation of mutations such that the probes cease to function (Kowald, Reference Kowald2015). However, evolution can be prevented by increasing replication fidelity – such a strategy minimizes waste – to arbitrary degrees using EDAC (Ellery and Eiben, Reference Ellery and Eiben2019a). This will eliminate the prospect of speciation into predator-prey ecologies in which self-replicating probes cannibalize other self-replicating probes or berserkers in which self-replicating probes seek out and destroy intelligent species.
The sheer utility of self-replication technology marginalizes the notion of that ETI will not develop this technology for fear of its potential dangers (Sagan and Newman, Reference Sagan and Newman1983). There are numerous reasons to send out self-replicating probes – reconnaissance prior to interstellar migration, first-mover advantage, insurance against planetary disaster, etc – but only one not to – indifference to information growth (which must apply to all extant ETI without exception). Self-replicating probes require minimal capital investment and represent the most economical means to explore space (Ellery, Reference Ellery2017), interstellar space included. In a real sense, self-replicating machines cannot become obsolete – new design developments can be broadcast and uploaded to upgrade them when necessary. Once the self-replicating probe is established in a star system, the probe may be exploited in various ways. The universal construction capability ensures that the self-replicating probe can construct any other device. These include monitoring devices to observe the evolution of intelligent species, direct communications with encountered intelligent civilizations to facilitate trade, directed panspermia seeding for planetary terraforming, construction of O'Neill colonies independent of planetary habitats for future worldships of colonists, etc. For example, panspermia offers the prospect of both seeding life and interstellar communication (Crick and Orgel, Reference Crick and Orgel1973). Biological cells can survive high shock impacts (Burchell et al., Reference Burchell, McDermott, Price and Yolland2014). Indeed, the panspermia concept suggests the prospect of exploiting microorganism DNA as a self-replicating message. This was explored in the bacteriophage фX174 that exploits Escherichia coli as its host (Yokoo and Oshima, Reference Yokoo and Oshima1979). Overlapping genes were selected for analysis and but no significant pattern was observed. Indeed, it is an open question whether self-replicating machines constitute a form of life themselves (Ellery, Reference Ellery2018a). It is far more likely that self-replicating probes will be used for colonization by robotically establishing infrastructure in preparation for subsequent worldships (Bond and Martin, Reference Bond and Martin1984). An intriguing prospect is that, given advances in 3D printing biological organs (Ellery, Reference Ellery2021a), the self-replicating probes could 3D print entire humans at a destination without the necessity for physical transport – the worldship concept may be rendered obsolete. Certainly, planetary habitats (Ellery and Muscatello, Reference Ellery and Muscatello2020; Ellery, Reference Ellery2021b) and their life support systems (Ellery, Reference Ellery2021c) may be manufactured through local resources and 3D printing technology. A human comprises almost entirely of 11 elements: O-65%, C-18.5%, H-9.5%, N-3.2%, Ca-1.5%, P-1.0%, K-0.4%, S-0.3%, Na-0.2%, Cl-0.2%, Mg-0.2% plus traces of 14 other elements – B, Cr, Co, Cu, F, I, Fe, Mn, Mo, Se, Si, Sn, V and Zn. Our industrial ecology already extracts all the major elements (emboldened) except P and K which may be extracted from KREEP minerals (Ellery et al., Reference Ellery, Lowing, Mellor, Conti, Wanjara, Bernier, Kirby, Carpenter, Dillon, Dawes, Sibille and Mueller2018) and several minor constituents (see Appendix Table A1). Although this in no way suggests that it would be straightforward to print organic material from these constituents (it wouldn't be), it does suggest at least that the basic constituents of a human being might recovered in-situ fairly trivially. Their organization, of course, is another matter entirely.
We further assume that appropriation of star system resources is ethical if resources are not being used (if no ETI is extant), biologically barren (tectonically inactive) or are negotiated through trade (if ETI is extant). The latter requires the ability to communicate through language. There are two theorems of interstellar trade that declare that interest must be computed relative to the trading planets rather than the travellers and that such interest rates between the planets converge (Krugman, Reference Krugman2010). Given that self-replicating probes trade locally with indigenous ETI, these factors are not relevant. The self-replication technology, or parts thereof, represents the most valuable trading commodity. Self-replicating probes provide the most economical means with which to colonize the entire Galaxy – it requires investment only in the initial probes sent from our solar system (built using self-replication technology developed on the Moon and elsewhere (Ellery, Reference Ellery2016a)). Self-replicating probes, of course, provide the most economic means for a civilization to transition from a Kardashev type II civilization into a Kardashev type III civilization (exploiting energy of the Galaxy ~4 × 1037 W) (Kardashev, Reference Kardashev1964) – this is the ultimate first-mover advantage. If we are an emerging KI civilization imminently developing self-replication technology, this suggests that the KI phase is short, only a few thousand years and the KII to KIII transition is longer at around a million years. We cannot comprehend the nature of a KIII civilization beyond that it should be detectable from megascale astroengineering constructions – no such evidence has been forthcoming through searches. If we apply the Copernican Principle (principle of mediocrity that we are typical in the Universe) which underlies the ETI hypothesis, we must assume that self-replication technology will be developed by ETI because this is exactly what we are doing.
Terran self-replicating probe payload
The self-replicating probe is based on the universal constructor, a machine that can construct any other machine (including itself) given the appropriate instructions and resources – John von Neumann was evidently strongly influenced by the ideas of Alan Turing (Burks and von Neumann, Reference Burks and von Neumann1966). The universal constructor requires four major components: (a) an autonomous factory that acquires and processes raw material into manufactured items that constitute the self-replicator (analogous to ribosomes); (b) an instruction program copier (analogous to DNA/RNA polymerase); (c) a controller that uses the instruction program for copying (uninterpreted) and for execution (interpreted) (analogous to protein-mediated gene expression); (d) instruction program that fully specifies A + B + C (analogous to DNA/RNA). von Neumann introduced his self-replicating machine model through cellular automata (CA) with specialized organs – neural cells, muscle cells and transmission cells. A physical representation of such cellular computing might comprise large arrays of simple computing units implementing CAs with simplicity, parallelism and local processing (Sipper, Reference Sipper1999). The self-replicating machine comprises two major systems – an universal computing machine and an universal kinematic machine within which the program copier may be subsumed into the kinematic machine and the program tape may be subsumed into the computer. Herein lie the clues to the critical components required for a kinematic self-replicating machine: (a) universal constructor that processes material through physical actuation on the environment; (b) universal computer that processes information through active physical electronics. The most basic component of the former is the actuator and of the latter is the active amplifier/switch. We are developing: (i) closed-loop extraterrestrial (lunar and asteroidal) resource-based industrial ecosystems from which to construct any kinematic machine (defined as a kinematic configuration of electric motors); (ii) 3D printing as a universal construction mechanism including (a) 3D printing electric motors (and so, the principle of self-assembly) that can be kinematically configured into suites of robotic machines; (b) 3D printing analogue neural network computers as a direct instantiation of the universal Turing machine based on vacuum tube amplifiers/switches. We contend that this tackles the key critical capabilities necessary to realize self-replicating machines subject to material, energy and information closure constraints (Ellery, Reference Ellery2020b).
Inspired by the RepRap 3D printer which can replicate many of its own plastic parts (Jones et al., Reference Jones, Haufe, Sells, Iravani, Olliver, Palmer and Bowyer2011), we suggest that completion of the self-replication process into a self-replicating 3D printer requires several lines of experimental development that we have been undertaking (it is not a necessary condition that 3D printing constitute universal construction as it may be supplemented by other FabLab kinematic machines (Tanaka, Reference Tanaka2001; Lipson, Reference Lipson2005) but it is a sufficient condition if we can demonstrate it):
(i) Multimaterial 3D printing to incorporate plastics, metals and ceramics: we have demonstrated the principle of using molten aluminium alloy with silicone plastics simultaneously (Fig. 1) which is a major step towards 3D printing metal/plastic in integrated components – indeed, this is precisely what is required in printing electronics and solar sails.
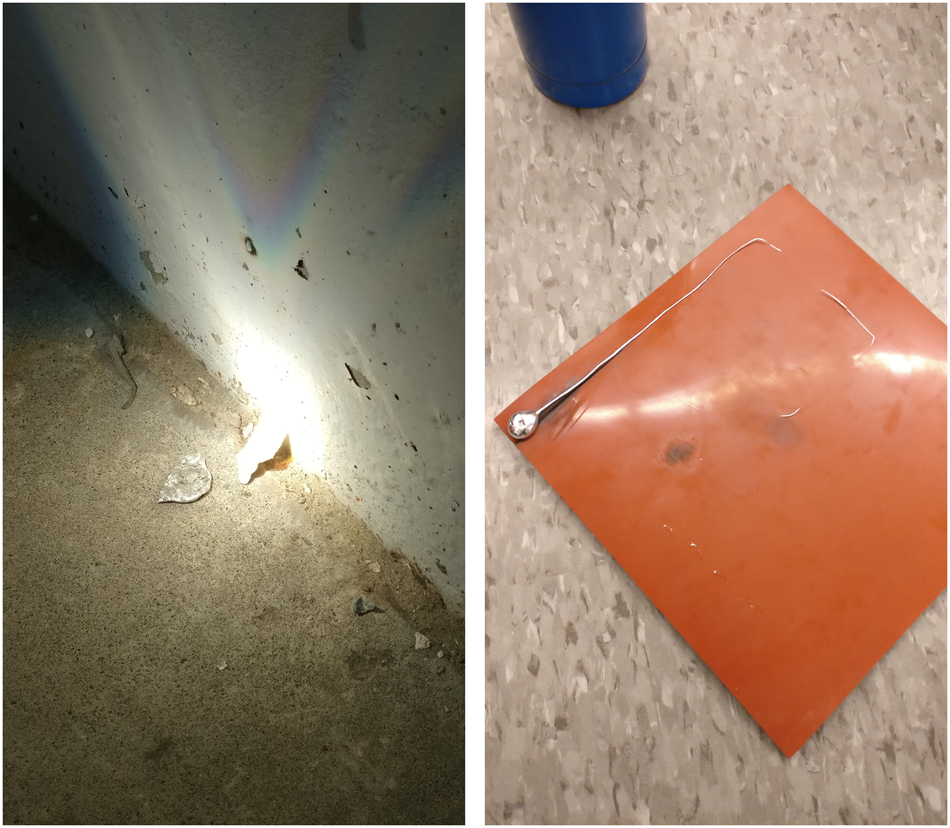
Fig. 1. (a) Fresnel lens melting of aluminium alloy; (b) molten aluminium directly on silicone plastic.
A custom 3D printer is under construction with three heads – a Fresnel lens-fibre optic bundle assembly for melting metal, a silicone plastic extruder and a milling head for surface finishing. A fourth three degree-of-freedom motorized ‘wrist’ head provides self-assembly capability.
(ii) 3D printing of electric motors: we have successfully demonstrated 3D printing of DC electric motors using a suite of 3D printing techniques (Elaskri and Ellery, Reference Elaskri and Ellery2020) (Fig. 2).

Fig. 2. (a) 3D printed prototype DC electric motor with wound coils (left) and off-the-shelf commercial DC electric motor (right); (b) exploded view of 3D printed DC motor with wound coils.
The current 3D printed motor is a prototype to demonstrate the principle of 3D printing complex mechanisms and subsequent versions will focus on printing coils and integrating the 3D printing processes to minimize manual assembly.
(iii) 3D printing of active electronics: we propose the adoption of vacuum tubes for computational electronics because it requires only a handful of materials and reagents with modest processing conditions (tungsten coated with quicklime/alumina cathode, high-temperature Kovar wiring, nickel control grid and anode and fused silica glass/ceramic enclosure) unlike solid-state processing which require stringent conditions (Ellery, Reference Ellery2022b). To obviate against the bulkiness of vacuum tubes, we have investigated the use of analogue neural network circuits which are Turing complete (Siegelmann and Sontag, Reference Siegelmann and Sontag1995) (Fig. 3).
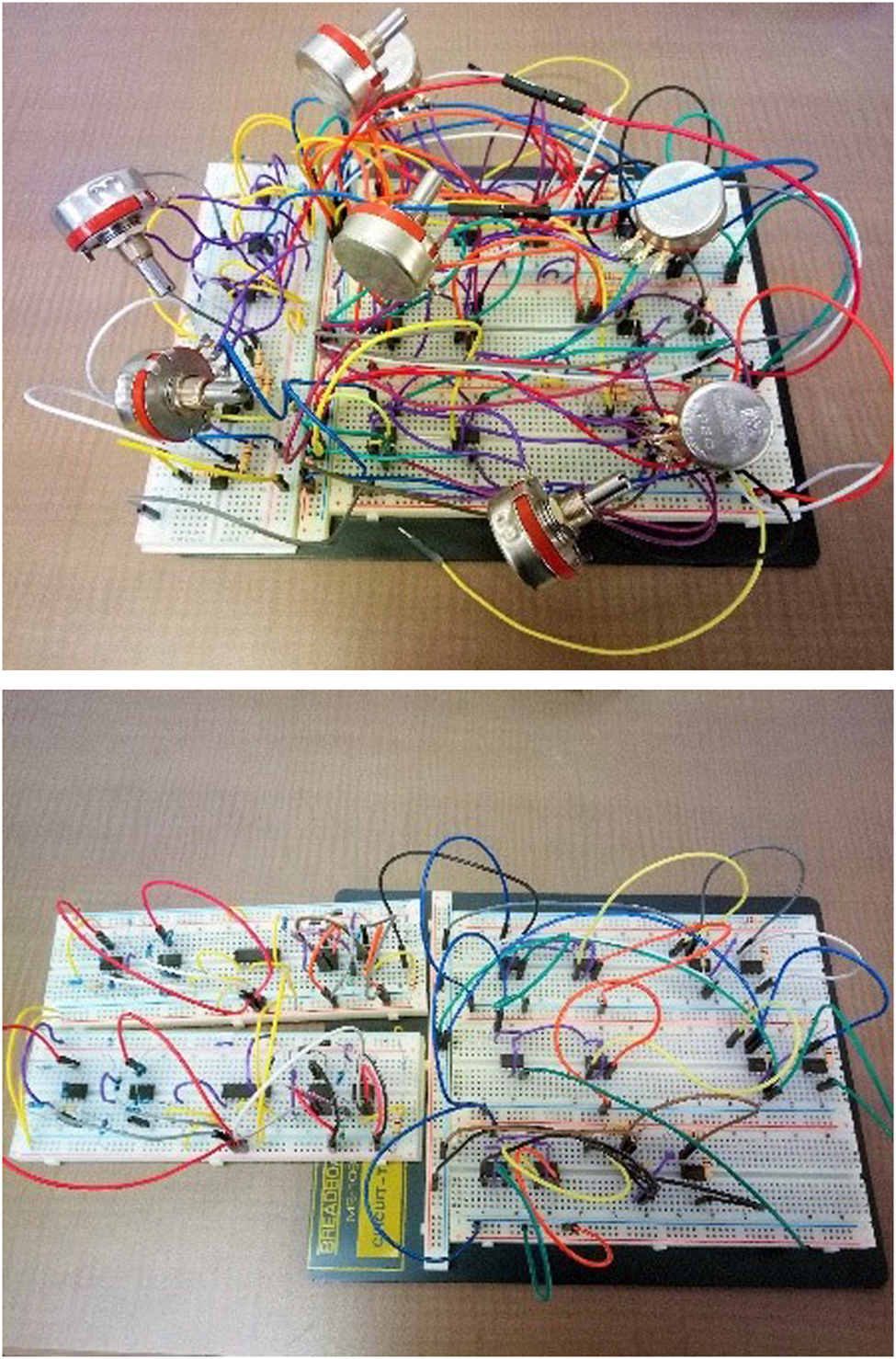
Fig. 3. Forward neural network and backpropagation physical analogue circuits.
Indeed, it has been argued that analogue neural network electronics may offer super-Turing capabilities (Siegelmann, Reference Siegelmann1995). Neocortical neurons that exhibit the multistability of digital flipflop circuits require the graded response of analogue circuits modulated by positive feedback gains in their recurrent connections (Hahnloser et al., Reference Hahnloser, Sarpeshkar, Mahowald, Douglas and Seung2000) reinforcing the utility of analogue circuitry. Digital behaviour in neural networks can be readily generated through McCulloch-Pitts neuron configurations. Optical neural networks are another possibility that offer promise for higher density interconnections with crossbar architectures, e.g. Hopfield net-type neural configurations (Hsu et al., Reference Hsu, Li and Psaltis1990). Neural nets can potentially be self-replicating – a self-replicating neural network version of a quine program has been shown to replicate itself by learning to output its own weights (Chang and Lipson, Reference Chang and Lipson2018). A quine is a program that prints itself – they comprise a program (phenotype) and data (genotype) in which the program uses the data in two ways: (i) genotype is transcribed into a new copy of the genome and (ii) genotype is translated into a phenotype (as established by von Neumann's self-replicator model). We have yet to 3D print vacuum tube components of our neural nets but they also provide the basis for radiofrequency devices such as magnetrons, klystrons and wigglers.
(iv) Self-assembly: we have married an earlier 3D printed electric motor without permanent magnets (except wire coils) with a 3D printed gearing system and two jointed panels to demonstrate simple self-assembly of structural panels (Ellery and Elaskri, Reference Ellery and Elaskri2019b) (Fig. 4).
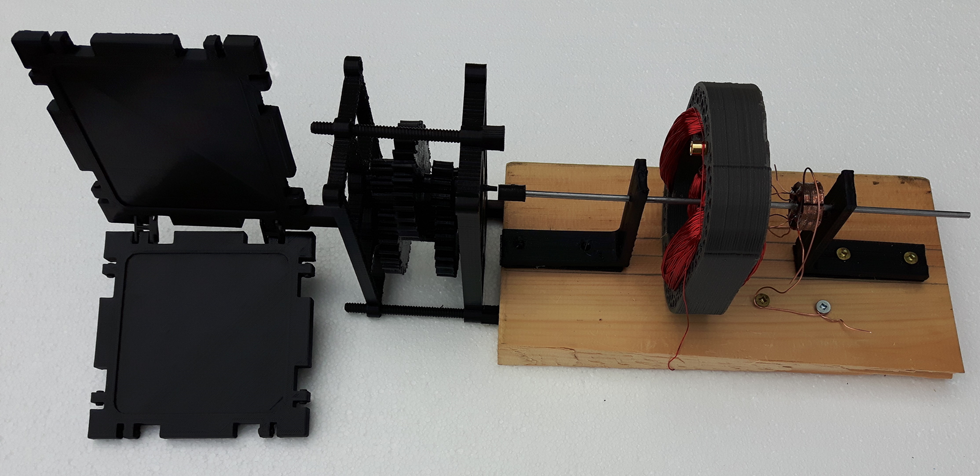
Fig. 4. 3D printed self-assembling system.
Next steps are to integrate the 3D printed motor and gearing into the hinge of the panels and then to construct a 3D printed reconfigurable robotics module. By implementing 3D printed motors and electronics, this would address the manufacturing plausibility of reconfigurable self-assembling modular robots such as those of Zykov et al. (Reference Zykov, Mytilinaios, Adams and Lipson2005).
(v) Preparation of 3D printer feedstock from raw materials: this is the realm of in-situ resource utilization (ISRU) of asteroid material. This is addressed below.
We need to source raw materials from extraterrestrial resources to 3D print our motors and electronics (we take the view from arguments outlined above that from 3D printed motors and electronics, omnia sequitur). Closure of energy, matter and information is a necessary condition for self-replication (though not for universal construction in general). Unlike terrestrial markets where capital costs such as mining, transport, processing, building, etc are spread amongst customers, all cost accounting in a self-replicator are self-contained within the self-replication cycle. Energy closure is well understood as EROI (energy return on investment) in that the energy cost cannot exceed the energy generated including accounting for energy consumed in mining raw materials, transport, processing and construction of the energy-generating machines (Hall et al., Reference Hall, Lambert and Balogh2013). One crucial factor is in the efficiency of conversion of environmental energy into electrical energy – for a self-replicating machine, high efficiency is necessary to ensure energy closure. Similar constraints on energy closure are imposed through material closure – the materials, parts and assemblies inventory must be constrained, favouring restricted suites of materials to minimize mining and chemical processing overheads, minimum machinery of production and assembly requirements – single-acid leaching, solid-state electrochemical processing and additive manufacturing with minimum waste are technologies that leverage material closure (Appendix). Information closure must similarly be constrained and traded with the material/energy cost of the information storage substrate. Indeed, our approach to power generation and storage through thermionic conversion and flywheels respectively (Ellery, Reference Ellery2021e) exhibits information compression by re-using technologies for vacuum tube-based computation and motors for actuation respectively – perhaps biological exaptation is similarly driven by the necessity for information efficiency driven by closure. These closure conditions are strict and unforgiving. For matter, energy and information closure, it is essential for the self-replicator to be as simple as possible to minimize the complexity of construction and maximize energy conversion efficiency. We must begin with an asteroidal materials inventory matched to our material demands. Hence, our demandite constitutes a minimal set of materials required to realize all the major functions required of a self-replicating machine (Table 2).
Table 2. Demandite materials list

We require several robotic processing facilities implemented by kinematic machines (which are, by definition, different kinematic configurations of electric motors): (i) mining robots for excavating regolith and minerals, (ii) beneficiation robots to physically separate desired minerals from gangue, (iii) electrochemistry robots to extract desired materials from minerals, (iv) fabricator robots to 3D print components from feedstock, and (v) assembler robots to assemble components into systems. The adoption of just-in-time protocols minimizes the requirement for warehousing while six sigma protocols with process recycling impose high-quality control standards. We have been exploring the use of genetic regulatory network approaches to controlling the industrial ecology (Ellery, Reference Ellery2021d). Onboard repair (such as wardens on the Daedalus starship) requires 3D printing (and supporting FabLab) facilities as a subset of self-replication – self-replication capability automatically incorporates self-repair implementing high reliability for free. All processing functions require computer controllers, power supplies and transport. Our industrial ecology minimizes waste in producing pure materials from minerals and gases from asteroidal and moon-type resources (Ellery, Reference Ellery2020c) (Appendix Table A1).
The most obviously useful materials are the M-type metal alloys. The carbonyl process can convert metal (Fe, Ni or Co) into its carbonyl form under different but modest conditions thereby separating and purifying them, all involving a FeS catalyst:
Fe(CO)5 ↔ 5CO + Fe (175oC/100 bar)
Ni(CO)4 ↔ 4CO + Ni (55oC/1 bar)
Co2(CO)8 ↔ 8CO + 2Co (150oC/35 bar)
This process is unlikely to yield a readily interpreted technosignature. Aluminium extraction from anorthite via silica and alumina is shown in the industrial ecology. We suggest that silicone plastics might be favoured over hydrocarbon plastics for their high durability and radiation tolerance in flexible electrical insulation and other applications. Silicone plastics are manufactured from syngas and silicon feedstock using metal/mineral oxide catalysts and recycled HCl (Rochow process) without yielding any waste products that are not recycled. It is well-known that olivine is unstable in the presence of water but both end members yield useful materials especially Si and SiO2 ceramic:
3Fe2SiO4 + 2H2O → 2Fe3O4 + 3SiO2 + 2H2
Mg2SiO4 + 2CH4 → 2CO + H2 + 2MgO + Si
Ferrite, silica, magnesia and silicon have useful applications. None of them yield waste products or specific signatures of such. Silicon/fused silica glass is useful in both forms and Mg metal may be released electrolytically. Pyroxene (nominally assumed to be enstatite) may be rapidly artificially weathered (natural weathering processes would be inconsistent with the incidence of olivine):
Mg2Si2O6 + HCl + H2O → Mg3Si4O10(OH)2 + H4SiO4 + MgCl2.nH2O
This yields silicic acid (from which silica is precipitated) and magnesium chloride as sources of silicon/fused silica glass and the clay talc which may be employed as a solid lubricant. The less common augite may be treated through the same process:
Ca(Fe,Al)Si2O6 + HCl + H2O → Ca0.33(Al)2(Si4O10)(OH)2.nH2O + H4SiO4 + CaCl2 + Fe(OH)3
This yields no waste product since the clay montmorillonite has widespread utility as the major component in bentonite drilling mud (but it would not be consumed so its existence would constitute evidence of artificial origin if discovered as extensive deposits) and CaCl2 is a universal electrolyte in the Metalysis FFC process. Enstatite can also be reduced by CH4 at 1600 °C to yield Si directly:
MgSiO3 + 2CH4 → Si + MgO + 2CO + 4H2
MgO can be reduced directly using the Metalysis FFC process. Our industrial ecology produces pure materials including pure oxides – all pure metal oxides can be reduced to arbitrarily pure metal through the Metalysis FFC process (Ellery et al., Reference Ellery, Mellor, Wanjara and Conti2021). The Metalysis FFC process involves the use of a sintered solid metal oxide powder (to be reduced to high purity metal while remaining in the solid state) cathode and a graphite anode at which oxygen is evolved (as carbon monoxide/dioxide). The molten CaCl2 electrolyte is not consumed in the process which is conducted at 900–1000 °C. The resulting high purity metal powder can be used as feedstock for metal additive manufacturing such as selective laser sintering/melting or electron beam additive manufacturing (Calignano et al., Reference Calignano, Manfredi, Ambrosio, Biamino, Lombardi, Atzeni, Salmi, Minetola, Iuliano and Fino2017). Electron beam processing constitutes another example of exaptation of thermionic devices. If the eroded graphite anode of the Metalysis FFC process is recycled and reconstructed using the Sabatier process followed by thermal cracking, the only byproduct of the Metalysis FFC process is oxygen which may be used as a reagent and/or evaporated off. The Metalysis FFC process can potentially be miniaturized – as a low-temperature process, aluminosilicate or fused silica glass microreactors with microfluidic channels connecting mixing chambers for the flow of reagents, heat-exchangers and dwell cavities offer high surface-to-volume ratios for efficient heat exchange (Dietrich et al., Reference Dietrich, Freitag and Scholz2005). Glass modules may be diffusion bonded under pressure at temperatures around the glass transition temperatures. These microreactors could potentially be incorporated as part of a self-replicating machine payload. The FFC process and 3D printing stage acts as the ‘knot’ of a bowtie processing architecture in which a wide range of inputs are funnelled through a small number of processes and then diverge into a wide range of output products – the bowtie architecture is characteristic of biological metabolism as a means to control complexity (Csete and Doyle, Reference Csete and Doyle2004). The key to any technosignature resides in the discarded waste products that are of little use and so remain unconsumed. The discovery of extensive waste deposits that would be unexpected naturally would invite artificial explanation. What are these waste materials? In this scheme, the industrial ecology recycles everything and the only extensive waste is in clays, many of which have use only during manufacture. For example, clays have catalytic properties but synthetic zeolites constructed from open-structured silica with Al3+ substitutions for Si4+ ions generating Bronsted acidity offer superior tailored catalysts. There is evidence of clays on asteroids (including their detection by OSIRIS-REx on the C-type asteroid Bennu) but these can be attributed to natural processes rather than technological ones. Although we have not considered all possible asteroid mineral types, we have considered the major ones and find that it is unlikely that there will be obvious signatures of artificial tampering with asteroidal material except through physical evidence of processing (which may be modified and distorted by subsequent impact gardening) or large-scale deposits of clays. Currently, there is no evidence of either that cannot be explained by natural processes. We tentatively suggest that the absence of evidence is indeed evidence of the absence of prior ET visitations in our solar system.
Self-replicating probe propulsion options
Our self-replication scheme was developed to support the construction of mechatronic systems and general spacecraft functions. We must now determine whether it can accommodate the requirements for the construction of interstellar propulsion systems as this is key to the feasibility of self-replicating interstellar probes. We are already embarking on interstellar probe technologies beyond the conceptual stage (McNutt et al., Reference McNutt and Wimmer-Schweingruber2011). The first consideration is scale – interstellar propulsion systems have a large scale. We assert that this presents a manageable issue if a modular approach is adopted. Provided a single module can be constructed by a single self-replicating unit, exponential population growth of the self-replicating system can rapidly accommodate any scale required. It remains only to indicate that all modules can be manufactured by a universal constructor. Any relativistic interstellar spacecraft requires high kinetic energy given by:

where β = (v/c). However, we assume non-relativistic speeds at 0.1 c which lie within near-term technological capabilities. Nevertheless, interstellar spacecraft are propelled at high speed through the interstellar medium. A 5 μm-sized interstellar silicate grain with a rest mass of 10−12 kg travelling at 0.1 c has a kinetic energy of 450 J which will require erosive shielding to combat. There are several potential interstellar propulsion techniques that dictate that interstellar travel at 0.1 c is feasible:
-
(i) Nuclear pulse rockets were adopted for the Orion spacecraft and the Daedalus starship concepts, the former ejecting hydrogen bombs that detonated against a pusher plate and the latter being a detailed design study for an interstellar starship fuelled from D/3He mined from gas giants (Bond et al., Reference Bond1978);
-
(ii) Bussard interstellar ramjet uses a large electromagnetic funnel to scoop up interstellar hydrogen at 1–2 atoms cm−3 ionized into a plasma for pp nuclear fusion catalysed by 12C (Bussard, Reference Bussard1960);
-
(iii) Ram-augmented interstellar rocket heats and accelerates collected hydrogen plasma using an electromagnetic field (Bond, Reference Bond1974);
-
(iv) Antimatter rockets release all the energy in matter but antimatter is manufactured in only very small quantities in particle physics laboratories (Forward, Reference Forward1982);
-
(v) Beam-propelled sails represent the most feasible approach based on near-term technology and do not require propellant (Forward, Reference Forward1984).
The 54 000 tonne Daedalus starship adopted nuclear fusion-based laser-pulsed pellet propulsion to reach 0.12 c for a flyby of Barnard's star within 50 light years (Bond et al., Reference Bond1978). The main components of the Daedalus nuclear pulse propulsion engine include: (i) reaction chamber of hafnium-tantalum-carbide (melting point of 4215 °C); (ii) excitation field coils of niobium-titanium; (iii) coil supports of titanium; (iv) laser ignition assembly comprising lasing medium and optics; (v) ignition charging circuits of copper; (vi) charging circuit supports of alumina; (vii) pellet injector including capacitors, superconducting magnets and coils for motors; (viii) thermal shielding of inconel/rhodium; (ix) cryogenic tankage of steel and its insulation. This approach requires a high fraction of challenging-to-extract materials. The most significant technological challenge for Daedalus was mining D/3He from Jupiter's atmosphere. 3He and D may be fused together to yield 4He with the production of large amounts of energy through nuclear fusion:
3He + D → 4He + H + 18.4 MeV
Although there is no damaging neutron production from this reaction, side DD reactions will occur in the plasma and generate some neutron flux. The D/3He fusion also requires a much higher ignition temperature than D/T fuel. D/T nuclear fusion is a future technology that is under development – it requires a 6Li blanket encased in Be within which T is bred by neutron bombardment – Li may be extracted from spodumene (LiAl(SiO3)2) minerals. For the D/3He reaction, the two components (especially 3He) may be mined from gas giants using aerostat balloons but this requires a complex set of facilities and nuclear pulse propulsion engine components require rare materials such as hafnium. Superconducting coils also require difficult-to-process materials – NbTi alloy is superconductive at cryogenic temperatures but perovskite cuprates are high-temperature type II superconducting materials, e,g. Ba-doped La2CuO4 (Fisk and Sarrao, Reference Fisk and Sarrao1997). Substitution of Ca and Sr doping also generates superconductivity as does the replacement of La with Na, K or Rb. There are many variants on these superconductors but they require complex chemical processing. Superconductors contain conducting CuO2 layers where each layer is separated from other layers. The London two-fluid model of total current through a superconductor is given by: J = (σ n + σ s)E where σ n,s = normal/superconducting channel conductivities, J = current density, E = electric field strength. The normal channel acts as resistance while the superconducting channel acts as an inductance. The requirement for these exotic materials eliminates (i)–(iii) though iron (II) selenide (FeSe) is a possible iron-based superconductor at 48 K when under pressure and intercalation. We consider antimatter drives too speculative. This leaves (v) as our choice of propulsion system. Beamed propulsion may also be applied to the ramjet (Matloff and Mallove, Reference Matloff and Mallove1988). Beam-powered sails were determined to be superior to nuclear fusion propulsion because (Benford, Reference Benford2017): (i) they can attain higher velocities; (ii) massive beamer propulsion is retained at the initial location rather than transported to the destination; (iii) laser physics is well-understood but nuclear fusion requires complex technological development.
Beamed propulsion
An interstellar sail may be unfurled as close to the Sun as possible to yield 2000 y long interstellar transits at cruise velocity $v_{i/s} = \sqrt \eta v_{{\rm peri}}$ and this is determined by the temperature limit at perihelion (Matloff, Reference Matloff2012). These long transit times favour power beaming. The beam of beamed interstellar propulsion may be laser or microwave. Beamed laser propulsion is based on the notion that photons exert pressure. These lasers will be of considerable size – the laser array may reside in Earth orbit or more likely sunward of the Sun-Earth L1 point balancing gravitation and solar pressure (similar to space-based geoengineering proposals (Ellery, Reference Ellery2016c)). CO2 lasers at 10.6 μm have efficiencies up to 10% while solar pumping of a Nd:Cr:YAG laser offers an efficiency of 35% (van den Donken, Reference van den Donken2019). A solar pumped laser using a Fresnel lens concentrating light onto a conical quartz rod to a grooved Nd:YAG lasing rod has been demonstrated for free-space laser communications (Zhao et al., Reference Zhao, Zhang, Gian, Je and Wang2019).
Free electron lasers (FEL) have efficiencies of 20% that may be further elevated if combined with solar pumping (Ellery, Reference Ellery2021e, Reference Ellery2022a) with the advantage that our self-replication scheme includes the construction of thermionic vacuum tubes and motor-based magnetic components. The FEL is an electron beam-based laser that generates bremsstrahlung radiation emitted by relativistic electrons passing through periodic magnetic fields (Madey, Reference Madey2014). The relativistic electrons are accelerated through a wiggler/undulator magnetic configuration, a periodic set of alternating magnetic poles transverse to the longitudinal beam path, generating an oscillating transverse magnetic field (Fig. 5) (O'Shea and Freund, Reference O'Shea and Freund2001; Krishnagopal et al., Reference Krishnagopal, Kumar, Maiti, Prabhu and Sarkar2004; Barletta et al., Reference Barletta, Bisognano, Corlett, Emma, Huang, Kim, Lindberg, Murphy, Neil, Nguyen, Pellegrini, Rimmer, Sannibale, Stupakov, Walker and Zholents2010).

Fig. 5. Free electron laser principle [CC-BY-SA 3.0 Frank Horst: https://en.wikipedia.org/wiki/Free-electron_laser#/media/File:FEL_principle.png].
These free electrons in a vacuum are forced to wiggle side-to-side in a sinusoidal pattern, acting as the lasing medium. This transverse acceleration of the electrons generates incoherent synchrotron radiation. Electrons are accelerated to relativistic speeds by an accelerator powered by klystrons. Partially-reflective mirrors at each end of the wiggler form a Fabry-Perot cavity to form standing electromagnetic waves. The transverse electric field component of synchrotron radiation interacts with the transverse electric current to generate a beat wave in synchrony with the electrons (ponderomotive gain). This causes longitudinal microbunching of electrons separated by one optical wavelength generating coherent radiation. Electron bunching with a bunching length much shorter than the radiation wavelength is the key. The wavelength of radiation is resonant and tunable through the electron energy or the undulating magnetic field:

where λ m = undulator wavelength, $\gamma = \left({1/\sqrt {1-\beta^2} } \right)$ = relativistic Lorenz factor, β = (v/c), K = (γλ m/2πr) = (eBλ m/2πm ec) = wiggler strength parameter, r = magnetic bending radius, B = applied magnetic field, m e = electron mass. The Lorenz factor upshifts the frequency of radiation. Peak FEL power is given by:

where E = electron energy, I p = peak current, I A = Alfven current, ɛ = electron emittance, β = betratron function of the beam envelope, JJ = Bessel function coupling factor for the undulator. The FEL mechanism is based on three processes: (i) electrons oscillate within the undulator and emit spontaneous radiation; (ii) radiation acts back on undulating electrons and bunches them; (iii) bunched electrons emit coherent stimulated radiation and amplify the electromagnetic waves. FEL is tunable over a wide frequency range from X-rays to microwaves offering GW peak powers by varying electron energy and magnetic field. However, EUV/X-rays cannot use resonant cavities so they must use long undulators (self-amplified spontaneous emission). High gain harmonic generation involves using a seed CO2 laser (photoinjector) in a short undulator comprising a modulation magnet followed by a higher harmonic magnet to cause microbunching for X-ray generation. FEL may also be used for high-intensity microwave generation (Orzechowski, Reference Orzechowski1990). There are several compact designs for terahertz (300 GHz-3 THz) FEL. A 20 MW free-electron laser operating at ~THz was driven by a THz laser that excites electron emission from a photocathode through a single-pass undulator in a desktop package (Huang et al., Reference Huang, Chen and Huang2008; Huang, Reference Huang2010). The Smith-Percell FEL is also a benchtop device that adopts a grating horn design (Bakhtyari and Brownell, Reference Bakhtyari and Brownell2003). A table-top FEL based on laser-accelerated electron bunches ~GeV incorporated miniature short-period magnetic undulators and miniaturized short-focal length focussing quadrupole magnets (Eichner et al., Reference Eichner, Gruner, Becker, Fuchs, Habs, Weingartner, Schramm, Backe, Kunz and Lauth2007). X-ray FEL can generate high-intensity X-rays with extremely short femtosecond pulses with angstrom wavelengths using long linear accelerators (Pellegrini, Reference Pellegrini2016; Seddon et al., Reference Seddon, Clarke, Dunning, Masciovecchio, Milne, Parmigiani, Rugg, Spence, Thompson, Ueda, Vinko and Wurth2017). A miniature X-ray tube of Kovar within which an array of carbon nanofibres on a nickel-plated tungsten wire constituting a high-energy electron field emission source indicates the viability of desktop X-ray devices (Haga et al., Reference Haga, Senda, Sakai, Mizuta, Kita and Okuyama2004). Indeed, the interaction of relativistic electron beams with electromagnetic waves can generate microwave, millimetre, infrared, ultraviolet and X-ray radiation (Granatstein et al., Reference Granatstein, Parker and Armstrong1999).
Microwave-propelled sails offer several advantages over light-propelled sails (Landis, Reference Landis1999a): (i) microwaves can be generated with much higher efficiency than laser beams; (ii) microwave phased arrays are established technologies on spacecraft; (iii) large microwave apertures are easier to construct than large laser apertures; (iv) microwave sails can be constructed from lightweight aluminium mesh because the perforations must be smaller than the wavelength of radiation. Perforated aluminium solar sails reduce the sail mass considerably (Matloff, Reference Matloff2003). Acceleration imparted to a microwave sail is given by:

where η = film reflectivity = 0.89, τ = film transmittivity≈0, α = film absorptivity = 0.1, ρ = areal mass density = 7 g m−2 for carbon fibre, A = sail area, c = light speed. The absorbed fraction must be radiated away from both sides of the sail:

where ɛ = sail emissivity = 0.6, σ = Stefan-Boltzmann constant. Hence,

For 9.8 m s−2 acceleration, T = 2000 K which requires a more temperature-tolerant sail material than aluminium such as tungsten (which is part of our self-replication scheme but is far rarer as a resource than aluminium) or ceramic. The Starwisp is a 1 km diameter sail comprised of thin wire mesh driven by microwave beam of 10 GW (Landis, Reference Landis1999a). The wire mesh can provide the synapses of neural network circuits, the nodes acting as neurons – this is a crossbar configuration. Given that volume is not a constraint for laser propulsion, we suggest a conventional FEL design. The basic components of FEL are: (i) accelerator that accelerates electrons relativistically; (ii) periodic magnetic undulator that forces electrons into a sinusoidal path; (iii) optical resonator that generates electron microbunching through resonant electron-radiation interaction.
The Forward lightsail concept adopts a 1000 km diameter Fresnel zone lens at 15 AU to focus 65 GW laser light to interstellar distances onto a 3.8 km diameter lightsail accelerated to 0.11 c for a flyby (Forward, Reference Forward1984). Deceleration however requires a 26 TW laser to intercept a 100 km diameter annulus sail. The Fresnel zone plate is a diffraction lens comprising a set of concentric rings of plastic (refractive index of 1.5) that alternate opaque and transparent zones. They are spaced so that diffracted light can constructively interfere at the required focus by adding a half-wavelength to the path difference between zones. We propose fused silica glass rather than plastic as this adds inertia to the Fresnel lens, requires only abundant silicate mineral resources and is compatible with our self-replication scheme. The radius of the nth zone is given by $r_n^2 \approx nf\lambda$ separated by spacing given by s n = r n − r n−1 where n = order and f = focal length. This effectively ensures that the spot size at α-Centauri is 100 km in diameter. Modular parabolic shells of carbon-fibre reinforced flexible silicone of ~10–20 m diameter have been proposed for large-aperture astronomical reflectors (Baier et al., Reference Baier, Datashvili, Endler, Roose, Stockman and Rodrigues2013). Another option for a ~10–20 m diameter Fresnel module is the planar photon sieve comprising a flat membrane filled with ~107 concentrically distributed ~μ-sized holes similar to a Fresnel zone plate in which the holes diffract light rather than grooves refracting light (Anderson, Reference Anderson2005). Hence, modules of a larger Fresnel lens could conceivably be constructed and assembled. Direct (optical) bonding is based on high van der Waal forces of adhesion F over a common surface area which requires extreme surface cleanliness and extremely low roughness <2 nm (Fischer et al., Reference Fischer, Gelinck, Semprimoschnig, van Munster and van der Heijden2015): F = (H/6πd 3) where H = Hamaker constant~10−20, d = 2 × asperity height. It is particularly suited to glass so this bonding might be exploited by a large Fresnel lens if contacting surfaces are extremely flat and smooth. During its construction, the Fresnel lens may also be employed as a Fresnel imager formed by an array of Fresnel diffraction lenses – a 30 m Fresnel lens could image Earth-sized planets within 30 light years to search for its next target. The closest analogue to the Fresnel zone lens is the solar shield concept for maintaining a 1000 km Fresnel lens sunward of the Sun-Earth L1 point to reduce solar flux to Earth by 2% to counteract global warming (Ellery, Reference Ellery2016c). Hence, the technology imposed by immediate geoengineering needs may facilitate interstellar travel.
Sailcraft is the envisaged mode for the $70 M Breakthrough Starshot project devised by Yuri Milner. A 100 GW phased array of lasers would be constructed in space or at high altitude to rapidly accelerate at 10 000 g a swarm of 1 m diameter ~1 g ultrathin light sail spacecraft with nano-electronic chip avionics to Alpha Centauri 4.3 light years away at 0.2 c. The key to this is to array modest lasers into a master oscillator power amplifier configuration. The DE-STAR (directed energy solar targeting of asteroids and exploration) system developed for asteroid deflection by laser ablation may be employed to accelerate wafer-like sailcraft to relativistic speeds (Lubin et al., Reference Lubin, Hughes, Bible and Johansson2015). A 10 km square phased array of modular lasers at 1.06 μm outputting 35 GW generates a 30 m diameter spot at a range of 1 AU. A photovoltaic array of similar size as the laser array provides power to the laser array. Laser diffraction is defined by the Rayleigh criterion:

where d t = transmitter diameter, d sail = sail diameter, R = spacecraft interstellar distance. Laser beaming can accelerate a 100 kg spacecraft with a 900 m diameter sail out to 30 AU up to 0.2 c – keeping the beam on the reflector after the beam area exceeds the reflector, the spacecraft velocity can be increased by $\sqrt 2$ towards 0.3 c. Radiation pressure force is given by:

where p = γm 0v = spacecraft momentum, $\gamma = \left({1/\sqrt {1-\beta^2} } \right)$, P = laser power, ɛ = 1 + r = emissivity for opaque sail, r = reflection coefficient, γ = relativistic factor. From this, time for laser beaming may be derived (Lubin et al., Reference Lubin, Hughes, Bible and Johansson2015):

where β = (v/c). At non-relativistic speeds, t = (p/F). A laser sail with a diameter of around 1 m may be accelerated to 0.25 c within a few minutes by a 100 GW laser array (Lubin and Hettel, Reference Lubin and Hettel2020). Directed lasers may also be used for Earth defence against asteroids through thermal ablation at 3000 K to deflect them (Lubin et al., Reference Lubin, Hughes, Eskenazi, Kosmo, Johansson, Griswold, Pryor, O'Neill, Meinhold, Suen, Riley, Zhang, Wash, Melis, Kangas, Motta and Brashears2016). However, a more responsive approach to asteroid mitigation has been proposed that exploits self-replication technology (Ellery, Reference Ellery2020a).
Lightsail
The light sail is a thin gossamer structure of wide cross section to capture laser light. Light sails are typically constructed from 2 μm thick Mylar/Kapton onto which a thin <0.1 μm reflective covering of aluminium is bonded. The minimum thickness for a sail for maximum absorption and reflection is dependent on the sail dielectric permittivity and electrical conductivity which varies with temperature and the frequency of incident electromagnetic waves – this equates to an aluminium thickness of <0.1 μm for light at 0.6 μm (Kezerashvili, Reference Kezerashvili2009). Typical dielectric permittivity values for aluminium on plastic are ɛ(1 − η) ≈ 4 and T max = 400 °C for Kapton. Aluminium density is 2.7 g cm−3 and aluminium is extracted from anorthite within our industrial ecology. Mylar/Kapton could be replaced with silicone elastomer which is our assumption here to minimize the materials inventory for self-replication to ensure closure. The use of high heat-tolerant silicone plastic can raise the maximum tolerable temperature to T max = 500–600 °C limited only by the melting point of aluminium at 660 °C. Dielectric thin-films such as alumina ~20–100 nm offer the higher temperature-tolerance but they have poorer reflectivity than metals (Landis, Reference Landis1999b). A thin protective layer of alumina can be applied by exposure of the aluminium sheet to oxygen and then polished to enhance its reflectivity. Higher temperatures still can be tolerated by tungsten film with its melting point of 3422 °C. Carbon fibre meshes are extremely fragile so are not considered suitable. Graphene offers hyper-thin, high-strength sail material which when combined with molybdenite to enhance its absorptivity gives 50% superior performance to a beryllium sail (Matloff, Reference Matloff2013). Graphene is a 2D sheet of sp2-hybridized carbon that be configured into 3D graphite, 1D nanotubes or 0D fullerenes. They are primarily manufactured through the Manchester mechanical exfoliation technique using cellophane tape applied to graphite (Allen et al., Reference Allen, Tung and Kaner2010). Solar sails are augmented by deployable booms which extend to unfurl the sail and provide rigidity. The sail typically requires a central hub from which four structural booms are deployed to support the sail. Structural rigging – nominally diagonal deployable carbon fibre-reinforced plastic booms add around 15% to the sail mass. The booms are typically comprised of two laminated polymer sheets bonded at the edges to form a tube. Wires of NiTi shape memory alloys are plausible for tensioning sails and indeed modulating this tension (Lynch et al., Reference Lynch, Jiang, Ellery and Nitzsche2016). Performance of the solar sail is determined by sail loading defined as the ratio of mass to area, σ(g/m 2) = (m s/A s) = (ρAt/A) = ρt and lightness number defined as the ratio of solar pressure to gravitation β = (σ c/σ) where σ c = critical sail loading = 1.53 g m−2 when solar pressure exceeds solar gravitation. Large sails can be assembled from smaller sail modules for ease of manufacture and handling – it is unclear if 3D printing can be implemented with extremely thin layers but we have demonstrated the viability of the deposition of aluminium onto silicone plastic (Fig. 1(b)). Thin <1000 μm PDMS (polydimethylsiloxane) membranes ~1 m in size have been 3D printed with features sizes ~0.1 μm using direct laser printing (Low et al., Reference Low, Chua, Ray, Mattia, Metcalfe and Patterson2017). This has the potential for coating of in-situ produced aluminium solar sails rather than Mylar. Large-scale high precision 3D printing will require sophisticated high precision self-localization and mapping (SLAM) capabilities which could be achieved through orbiting interferometric laser-based GPS-type constellations if assembly is performed at Lagrangian points. 3D structures may be implemented in the payload as flat-pack components that unfold. Self-folding machines such as mobile robots may be configured initially as flat sheets with embedded circuits that activate elastic flexures of shape memory material around thinned hinges into unfolded 3D structures (Felton et al., Reference Felton, Tolley, Demaine, Rus and Wood2014).
The rigidity of the sail can be ensured through various means (Cassenti et al., Reference Cassenti, Matloff and Strobl1996)– the heliogyro comprises long tapes spinning like helicopter blades; canopy sails are analogous to parachutes and require them to be convex in the direction of the laser for stability; hollow body solar sails are pressurized cylinders – these all require sufficient stiffness to tolerate instabilities. It has been demonstrated that PDMS membranes can be treated to increase their stiffness through selective polymeric photo-cross-linking (Femmer et al., Reference Femmer, Kuehne and Wessling2014). There have been several successful solar sail demonstrations, e.g. IKAROS (2010), NanoSail-D2 (2011), LightSail-1 (2015) and LightSail-2 (2019). The NanoSail-D was a 20 m × 20 m square solar sail test system stowed in a cubesat that successfully flew and deployed in 2010 (Johnson et al., Reference Johnson, Young, Montgomery and Alhorn2011). Lightsail-2 was a 32 m × 32 sail that demonstrated controlled sailing with orbital manoeuvres. The momentum and kinetic energy imparted to the sail at relativistic speeds assuming perfect reflectivity is given by:


Beamed laser propulsion is limited by diffraction-based divergence given by:

where λ = laser wavelength, d = optics diameter. The laser spot diameter d increases with range until it fills the sail diameter d s at range R c beyond which the intercepted laser power drops as 1/R 2 (Moeckel, Reference Moeckel1972):

Photon pressure on the sail is given by:

where Pr = received beam power density, $P_r = ( 4P_t/\pi d_s^2 )$ for R < R c and P r = (P r/(R/R c)2) for R > R c. Acceleration of the sail imparted by photon momentum is given by:

where A = sail area, r = reflectivity. If we assume that the mass of the sail dominates the total mass:

The Δv imparted is given by:

Optimal sail thickness is 20–50 nm. For a radiation-cooled sail, the maximum received power density is determined by the material temperature (in this case, aluminium/silicone plastic):

Hence,

where ɛ = emissivity
The interstellar sail is a structure of magnitude but there is no physical reason why it cannot be constructed by leveraging the production capacity of self-replicating machines which can exponentially achieve scale from a population of modules.
Electric and magnetic sails
Deceleration of the sail can be achieved by detaching an inner segment of the sail that reflects the laser beam from the outer ring to the inner section to decelerate it (Forward, Reference Forward1984). In Forward's original conception, an inner 30 km diameter disc sail is surrounded by a 100 km diameter ring sail launched by a 7.2 TW laser to impart an acceleration of 0.05 ms−2 to a cruise velocity of 0.2 c. For deceleration, the original laser is upgraded to a 26 TW laser beam. The inner section detaches, reverses its reflective direction (so it reduces the effect of direct laser flux) and positions itself to the focus of the ring sail. The ring sail is accelerated at 0.2 m/s2 past the destination while reflecting impinging laser light onto the inner section, decelerating it at 2.0 m s−2 for one year. The mission duration to α-Centauri is 41 years. Alternatively, magnetic and/or electric sails may be employed to decelerate the sail (Perakis et al., Reference Perakis, Schrenk, Koop and Losekamm2016). The electric sail comprises extended charged conducting tethers which are spun up around its axis of symmetry. A thermionic electron gun is employed along the spin axis to generate an electric field which interacts with interstellar ions with a Coulomb drag force given by (Perakis and Hein, Reference Perakis and Hein2016):

where N = number of tethers, L = tether length, Vs = sail voltage, e = electronic charge, rw = wire radius, $\lambda _D = \sqrt {( \varepsilon _0k_BT_e/n_{ion}e^2) }$, ɛ 0 = electrical permittivity of free space, kb = Boltzmann's constant, Te = electron temperature = 8000 K. The power requirement is given by:

The magnetic sail comprises a superconducting coil through which a current generates a magnetic field moving at velocity through ions of the interstellar plasma which are deflected (Andrews and Zubrin, Reference Andrews and Zubrin1990). The superconducting loop may be deployed from a drum out to ~102 km and hoop stresses force a circular shape. The magsail generates high deceleration force on the magnetic sail acting as magnetic drag (Perakis and Hein, Reference Perakis and Hein2016):

where m p = proton mass, n ion = number density of ions = 0.03 cm−3, μ 0 = permeability of free space, I = coil current, r s = sail radius, v = sail speed. The mass of ablative shielding is required due to collision with ions:

where $( {\rm d}m_{{\rm shield}}/{\rm d}t) = ( A_{{\rm coil}}m_pn_{{\rm ion}}/{\rm \Delta }H) ( \beta c^3/\sqrt {1-\beta ^2} ) \left({( 1/\sqrt {1-\beta^2} ) -1} \right)$, t = deceleration time, A coil = coil cross section, ΔH = enthalpy of vaporization of ablative material (e.g. graphite). Smaller magsails are feasible using mini-magnetospheric magsails in which particle beams (propellant) are reflected by a magnetic field (Landis, Reference Landis2001). A particle beam may be generated by an accelerator (Andrews, Reference Andrews2003). For example, the betatron is a compact cyclic particle accelerator based on a transformer and a torus-configured vacuum tube acting as the secondary coil. AC is applied to the primary coils which through Faraday magnetic induction H = (1/2)(ϕ/πr 2) accelerates electrons around the torus with orbital radius r enclosing a magnetic flux φ. The betatron can generate high-energy electron beams up to 300 MeV which on striking a metal plate can generate X-rays. Nanophotonic acceleration of charged particles with efficient transfer of momentum energy from laser to particles may also be achieved using nanophotonic dielectric structures comprised of rows of 3 μm high pillars with separations of 225 nm (Shiloh et al., Reference Shiloh, Illmer, Chlouba, Yousefi, Schonenberger, Niedermayer, Mittelbach and Hommelhoff2021). A phase focussing method involves minimal particle loss allowing reduction of the size of particle accelerators by orders of magnitude. The combination of deploying a magnetic sail at high velocities and an electric sail at lower velocities yields more rapid deceleration from 0.05 c to interplanetary velocities (29 years) than either alone (40 years for magnetic braking only and 35 years for electric braking only) (Perakis and Hein, Reference Perakis and Hein2016). In both cases of electric and magnetic sails, the required electromagnetic devices are incorporated into our self-replication scheme.
Conclusions
We are currently developing self-replicating machines – although there remain challenges associated with the large scale associated with interstellar travel, our current self-replication scheme ensures that the fundamental technologies can be developed in short order. This suggests that if we apply the Copernican principle, ETI, if they exist, should have developed self-replicating probes. There is no observational evidence of large structures in our solar system, nor signs of large-scale mining and processing, nor signs of residue of such processes. Our current terrestrial self-replication scheme with its industrial ecology is imposed by the requirements for closure of the self-replication loop that (i) minimizes waste (sustainability) to minimize energy consumption; (ii) minimizes materials and components manufacture to minimize mining; (iii) minimizes manufacturing and assembly processes to minimize machinery. Nevertheless, we would expect extensive clay residues. We conclude therefore that the most tenable hypothesis is that ETI do not exist. However, this does not invalid SETI searches of any kind – indeed, it requires them in a Popperian sense to attempt to disprove the hypothesis (Ellery, Reference Ellery2003). The self-replicating machine concept has profound implications: (i) for the long-term growth of humanity as a cosmological phenomenon (Barrow and Tipler, Reference Barrow and Tipler1998) in transitioning from a Kardashev type 1 civilization (that consumes energy on a planetary scale) to a type 3 civilization (that consumes energy on a galactic scale) (Kardashev, Reference Kardashev1997)), (ii) for our relationship with artificial intelligence required for a self-replicating machine (Bostrom, Reference Bostrom2014), (iii) for the implications of self-replication technology for the non-existence of extraterrestrial intelligence and in providing humanity with first mover advantages in interstellar exploration (Ellery, Reference Ellery2019b). These aspects are more speculative but nevertheless suggest that the self-replicating machine is the ultimate machine affording unchallenged cosmological power to the human species over the longest term.
Appendix
Table A1. Industrial ecology that supports self-replication process (emboldened oxides are feedstock for the Metalysis FFC process)
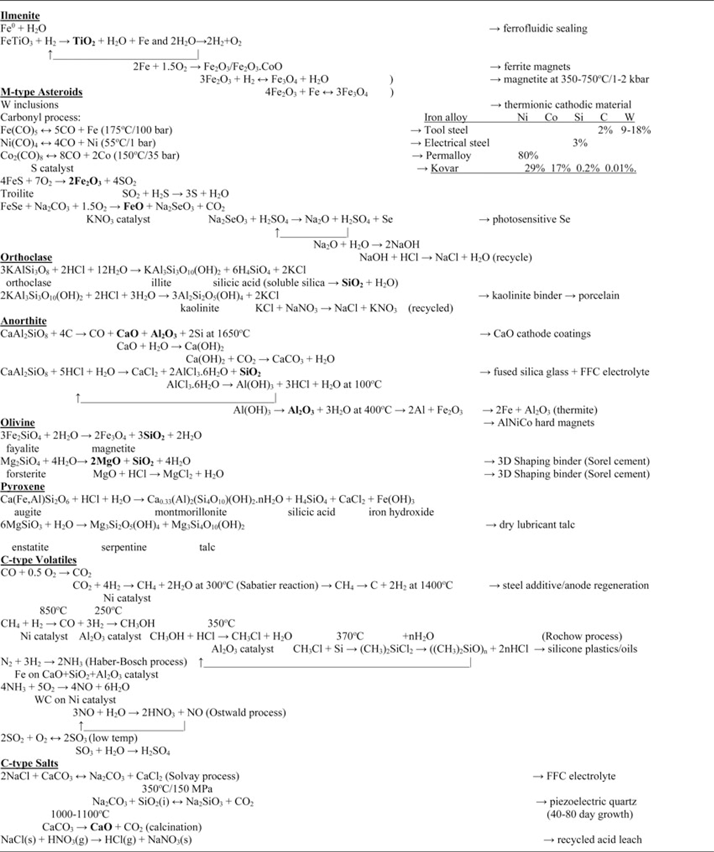