Introduction
In developed countries, population ageing is a major demographic trend and will remain so in the next decades. Accordingly, health issues concerning the elderly have increased in importance and have entailed an ever-growing level of economic costs. Among these health issues, loss of memory and alterations in behaviour associated with declining brain function have a large impact on society and the economy. These changes with ageing are also key symptoms of degenerative brain diseases, such as Alzheimer’s disease (AD) and other dementia forms( Reference Freemantle, Vandal and Tremblay-Mercier 1 ). Furthermore, there are many forms of chronic debilitating brain disorders besides dementias. It has been claimed that in the next years the impact of the wide array of brain disorders will possibly surpass that of CVD and cancer taken together( Reference Abdin-Sobocki, Jönsson and Wittchen 2 ). Therefore, it is of paramount importance to achieve a deeper knowledge of the conditions for optimal brain function and cognition. It is important to point out that prevention is more effective than treatment in curbing the societal and economic costs. Taking this into account, nutrition may have a very significant role for this objective. In fact, there are aspects associated with nutrition that affect the risk of cognitive function decline and neural and psychiatric outcomes.
DHA, one of the most important marine n-3 PUFA, may have a strong influence on brain health( Reference Freemantle, Vandal and Tremblay-Mercier 1 ). Indeed, consumption of larger amounts of n-3 PUFA, particularly DHA, appears to reduce the risk of depression( Reference Hibbeln and Davis 3 ), including postpartum depression, bipolar disorder (manic depression), schizophrenia, and mood and behaviour disorders( Reference Milte, Parletta and Buckley 4 ). It has also been hypothesised a connection between DHA in the diet and in the nerve cell membrane and the risk of dysfunction of the central nervous system in the form of anxiety( Reference Ross 5 ), irritability, susceptibility to stress( Reference Hellhammer, Hero and Franz 6 ), dyslexia( Reference Stordy 7 ), stereotypic behaviour, aggressiveness( Reference Gow and Hibbeln 8 ), reduced learning capacity( Reference Milte, Parletta and Buckley 4 ), impaired memory and cognitive functions, and extended reaction times( Reference Stonehouse, Conlon and Podd 9 ).
The present review will focus on the role of DHA in the nervous system and cognitive function as well as in the prevention of cognitive decline associated with ageing. The state-of-the-art in these scientific areas of research will be analysed taking into account the DHA chemical form (Fig. 1), that is, the wider chemical structure where DHA is bound (TAG, NEFA, ethyl ester and phospholipid (PL)) and its effects on DHA bioaccessibility and bioavailability.
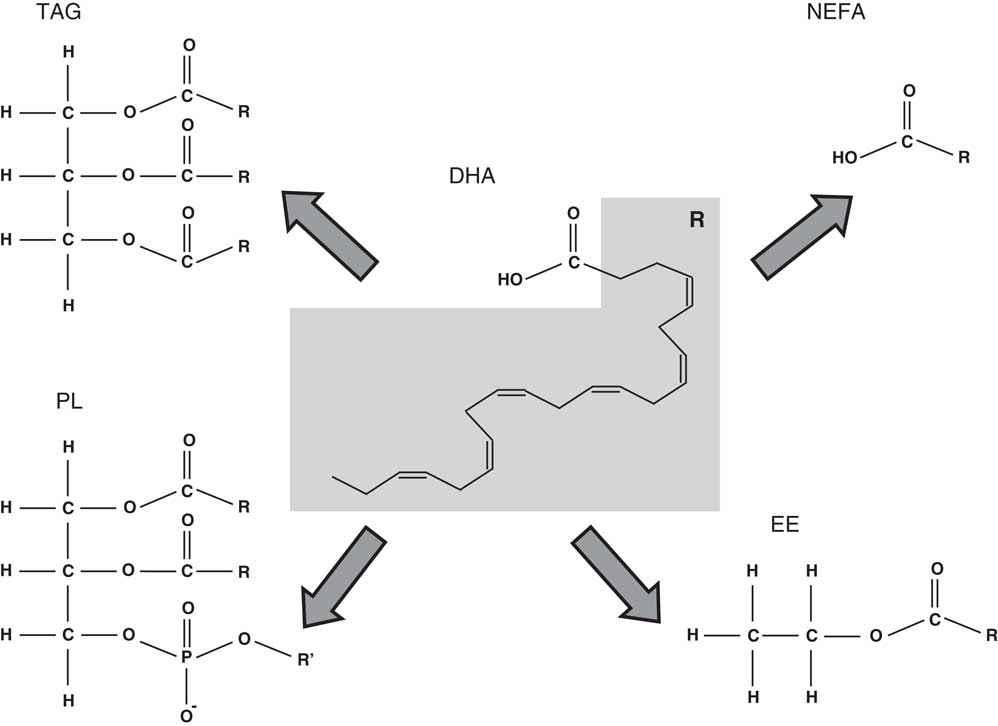
Fig. 1 Chemical structure of the different chemical forms in which DHA may be found. PL, phospholipid; R’, choline, serine, ethanolamine, etc.; EE, ethyl ester.
DHA and its role in cognitive ageing: evidence discussion
Ageing and the cognitive function decline associated with it pose a great challenge to societies in developed countries. The loss of cognitive abilities may vary immensely in kind and degree and may affect not only elderly, but also middle-aged individuals. In the most serious situations, pathologies are identified. As aforementioned, there are many forms of chronic debilitating brain disorders, nutrition being a possible key to the prevention and mitigation of some of their effects. DHA plays an important role in ensuring healthy ageing, by possibly thwarting macular degeneration, AD and Parkinson’s disease, and other brain disorders at the same time as enhancing memory and strengthening neuroprotection in general. A reduced level of DHA in the blood is associated with cognitive decline during ageing( Reference Mohajeri, Troesch and Weber 10 ). An overview of the various studies concerning the impact of DHA on AD (and other cognitive decline situations) as well as on healthy individuals is presented in Table 1.
Table 1 Overview of some significant intervention and observational studies concerning the effects of DHA on the cognitive decline due to ageing

AD, Alzheimer’s disease.
There are several important studies correlating dietary DHA and cognitive function ageing effects. These studies relate to different human populations that can be healthy or presenting mild cognitive impairment (MCI)/AD/other cognitive function disorders.
Some interesting studies, either observational or randomised controlled trials (RCT), have been carried out with healthy populations( Reference Muldoon, Ryan and Sheu 11 – Reference Yurko-Mauro, McCarthy and Rom 13 ). For instance, in a community-dwelling cohort, levels of α-linolenic acid (ALA), EPA and DHA were assessed in serum PL of volunteers not taking fish oil supplements( Reference Muldoon, Ryan and Sheu 11 , Reference Wurtman, Cansev and Sakamoto 14 ). It was found out that only the associations between serum PL DHA and non-verbal reasoning and working memory remained after adjustment for participant education and vocabulary. Moreover, DHA increased cognitive performance in an RCT involving mentally healthy individuals older than 55 years( Reference Yurko-Mauro, McCarthy and Rom 13 , Reference Yurko-Mauro 15 ). Daily supplementation of 900 mg of algal (Schizochytrium sp.) DHA for 24 weeks was associated with significantly lower paired associative learning errors than the placebo case. Similar results were attained by an RCT study( Reference Witte, Kerti and Hermannstädter 12 ) on executive functions and neuroimaging in a group of healthy subjects whose age ranged between 50 and 75 years. The authors registered a benefit in executive function including verbal fluency. They also found alterations in white matter microstructural integrity (interpreted as beneficial) as well as increases in gray matter volume in the frontal, temporal, parietal and limbic areas( Reference Witte, Kerti and Hermannstädter 12 ). In a large cohort of Chinese adults (average age of 65 years; part of the Singapore Longitudinal Aging Studies (SLAS)), daily consumption of fish oil supplements was associated with higher Mini-Mental State examination scores and a lower risk of cognitive decline over a 1·5-year period( Reference Gao, Niti and Feng 16 ).
All these studies involving healthy subjects have some drawbacks. In fact, while the study by Witte et al. ( Reference Witte, Kerti and Hermannstädter 12 ) involved a very small population (n 65), the SLAS did not control the level of DHA intake. Therefore, both studies’ conclusions are weakened by these shortcomings. The study by Yurko-Mauro et al. ( Reference Yurko-Mauro, McCarthy and Rom 13 ) seems better designed and more robust than others and clearly points to positive effects of 0·9 g DHA/d. However, the study by Velho et al ( Reference Velho, Marques-Vidal and Baptista 17 ) did not find an effect of any PUFA on cognitive function. Hence, though studies on healthy elderly seem to point to a beneficial net effect of DHA on cognitive ageing, evidence is still far from convincing, further studies being required.
For individuals with MCI, some interesting studies( Reference Chiu, Su and Cheng 18 – Reference Lee, Shahar and Chin 20 ) have also been carried out. The evidence has been recently reviewed( Reference Salem, Vandal and Calon 21 ). Namely, the Memory Improvement After DHA Study (MIDAS) demonstrated that DHA may be advantageous in healthy adults with a mild memory complaint( Reference Yurko-Mauro, McCarthy and Rom 13 ), thereby emphasising the role of prevention. Another study, Lee et al. ( Reference Lee, Shahar and Chin 20 ), has reported a benefit for several measures of memory function in a group of elderly patients with MCI. Furthermore, in an RCT study, DHA provided benefit for several measures of memory and attention score in subjects with MCI( Reference Kotani, Sakaguchi and Warashina 19 ). However, in a study by Daiello et al. ( Reference Daiello, Gongvatana and Dunsiger 22 ), it was concluded that a causal effect of fish oil supplement use on cognition was not proven, further research being warranted. On the other hand, in the Italian Longitudinal Study on Aging, there was no significant effect on the protection against the development of MCI( Reference Solfrizzi, Colacicco and D’Introno 23 ). These latter studies oppose the view that benefits of DHA are easier to detect during ageing whenever there is some MCI or memory complaint or possibly if an individual is under the influence of some physical or mental stressors( Reference Salem, Vandal and Calon 21 ).
A critical appraisal of these studies relating to MCI raises doubts about the beneficial action of DHA on MCI onset and development. The more positive results were attained in studies with small populations (thirty-six or lower)( Reference Chiu, Su and Cheng 18 – Reference Lee, Shahar and Chin 20 ). The studies with larger populations (186 or higher) did not show significant results( Reference Daiello, Gongvatana and Dunsiger 22 , Reference Solfrizzi, Colacicco and D’Introno 23 ), but they were observational studies where high DHA intakes were not tested by a significant share of the subject set. Accordingly, the protective role of DHA in MCI is still dubious.
Nevertheless, the effects of DHA on cognitive ageing, MCI and dementia other than AD have been more supported by evidence than those on AD. Whereas, according to some authors, DHA improved cognitive abilities in individuals with MCI, the effects on AD patients were not obvious( Reference Mohajeri, Troesch and Weber 10 , Reference Chiu, Su and Cheng 18 , Reference Kotani, Sakaguchi and Warashina 19 ). Indeed, it has been mentioned that once AD is clinically evident, supplementation trials show no significant effect of DHA on AD( Reference Cunnane, Chouinard-Watkins and Castellano 24 ). Nevertheless, several prospective observational studies clearly point to a protective effect of higher DHA intake against risk of AD( Reference Morris, Evans and Bienias 25 , Reference Schaefer, Bongard and Beiser 26 ). Hence, prevention is more effective than treatment. This assessment of the observational studies has been shared by different review papers( Reference Cunnane, Chouinard-Watkins and Castellano 24 , Reference Cunnane, Plourde and Pifferi 27 , Reference Fotuhi, Mohassel and Yaffe 28 ). On the other hand, there are other observational studies that did not find any association between DHA intake and AD risk( Reference Devore, Grodstein and van Rooij 29 ). A meta-analysis reviewing the association of n-3 PUFA and DHA with AD incidence found no significant evidence( Reference Wu, Ding and Wu 30 ). However, in some populations, such as the Dutch( Reference Devore, Grodstein and van Rooij 29 ), fish consumption and DHA intake are quite low( Reference Cardoso, Bandarra and Lourenço 31 ), thus entailing statistical problems given the very low number of subjects with a DHA intake high enough to reduce AD incidence. Furthermore, another interesting study( Reference Quinn, Raman and Thomas 32 ), the Alzheimer’s Disease Cooperative Study, found out that DHA did not produce any benefit in the primary outcomes, but observed a benefit for cognitive score in ApoE4 allele-negative patients. Indeed, AD patients in this group had a significantly lower decline in the Alzheimer’s Disease Assessment Scale score over 18 months with a daily dosage of 2 g of DHA.
A comparison between the studies concerning DHA and AD (Table 1) shows that some studies do not have a representative population sample( Reference Chiu, Su and Cheng 18 , Reference Kotani, Sakaguchi and Warashina 19 ) and, as such, their significance is quite weakened. The Dutch study( Reference Devore, Grodstein and van Rooij 29 ) seems much more solid and representative. The other observational studies are more modest and show beneficial DHA effects on AD that were not found in the Dutch study( Reference Morris, Evans and Bienias 25 , Reference Schaefer, Bongard and Beiser 26 ). The RCT study by Quinn et al. ( Reference Quinn, Raman and Thomas 32 ) may harbinger a new generation of studies that are supported by a priori genetic analysis. This will provide much more insight. Meanwhile, evidence connecting DHA intake and containment of AD progression after its onset is very insufficient.
Whether healthy or MCI or AD subjects, the assessed studies do not provide incontrovertible outcomes. It is possible that the beneficial effects of DHA concern solely AD and MCI prevention and be entirely absent once clinical conditions, especially if severe (AD), are already present. But, results do not allow for such conclusion. Perhaps, more importantly, future studies should always separate population groups in accordance to their genes, since some causal links may only occur in specific genotypes. Studies encompassing larger populations and longer periods are also warranted.
DHA and its role in cognitive ageing: dose–response and mechanisms
The calibration of the DHA dosages for achieving a significant response is another issue that requires new studies. Some of the daily DHA dosages are quite high. For instance, in order to achieve 2 g/d of DHA, a daily meal of 130 g of Atlantic mackerel or 120 g of Atlantic salmon may be required (Table 2). Therefore, it would be difficult to achieve such high DHA intakes without supplements. Moreover, in future RCT, the issue of DHA bioavailability (see the ‘Dietary sources of DHA, bioaccessibility and bioavailability’ section) should be taken into account – for instance, the same DHA dosage given to different individuals can lead to different levels of bioavailable DHA as a result of changes in the functioning of the digestive system due to age and disease – and a better selection of DHA supplements (including chemical binding form) should be ensured.
Table 2 Average DHA content (mg/100 g) in different marine sources, not subjected to any culinary process( Reference Bandarra, Calhau and Oliveira 112 , Reference Peinado, Girón and Koutsidis 113 , Reference Makri, Bellou and Birkou 130 – Reference Taoka, Nagano and Okita 132 )

* For microalgae and seaweeds, DHA contents are given in mg/100 g DM.
† For microalgae and seaweeds, richness was assessed assuming 20% DM as is usually the case in seafood.
For those studies involving AD patients, it has been observed that though DHA intake is low, brain DHA levels are frequently similar to the controls, thus suggesting that low DHA intake leads to low plasma DHA, but does not necessarily decrease brain DHA( Reference Cunnane, Chouinard-Watkins and Castellano 24 ). Accordingly, these authors have claimed that animal models involving dietary n-3 PUFA deficiency in order to deplete brain DHA may not be adequate in AD research. Moreover, it has been claimed that the fatty acid (FA) profile of plasma total lipids is not an appropriate measure of DHA status in AD because it seems to mask lower DHA in plasma PL offset by higher DHA in plasma cholesteryl esters( Reference Corrigan, Van Rhijn and Ijomah 33 , Reference Cunnane, Schneider and Tangney 34 ). Hence, it is of paramount importance to analyse DHA in each lipid class. AD has been associated with changes in plasmalogen choline as well as in the amount of DHA found in different PL( Reference Igarashi, Ma and Gao 35 ).
In the mechanistic analysis of the link between DHA and cognitive function, it should be noted that DHA is by far the main n-3 PUFA present in the brain – its content within brain FA is 12–15 %( Reference Whelan 36 ) – where it is predominantly located in neuronal membranes of the grey matter, especially in synapses( Reference Cunnane, Chouinard-Watkins and Castellano 24 ). In addition, the brain FA-binding protein preferentially binds DHA (and other n-3 PUFA)( Reference Hanhoff, Lücke and Spener 37 ), leading to higher levels of DHA incorporation in the molecular structures of the membranes( Reference Storch and Thumser 38 ).
DHA is supplied to the central nervous system by the liver, where DHA attained from food is taken up and distributed to other organs( Reference Scott and Bazan 39 ). Besides, though there is evidence suggesting the expression and functional role of FA transporters at the blood–brain barrier( Reference Mitchell and Hatch 40 ), DHA can reach the brain by simple diffusion through this barrier( Reference Hamilton and Brunaldi 41 ). On the other hand, the dietary level of α-linolenic acid (ALA; 18 : 3n-3), a precursor of DHA, does not correlate well with the level of DHA in the human body, making it advisable, for instance, to supplement the nursing mother’s diet with DHA( Reference Ratnayake and Hollywood 42 ). Furthermore, it should be remarked that plasma or erythrocyte DHA does not correlate well with DHA in the brain cells( Reference Cunnane, Chouinard-Watkins and Castellano 24 , Reference Beydoun, Kaufman and Satia 43 – Reference Manzato, Roselli della Rovere and Zambon 45 ).
DHA is highly enriched in the PL of the synaptic plasma membrane and synaptic vesicles( Reference Glomset 46 ). Regarding this issue, it is worth analysing the pathways leading to the synthesis of some important PL. Phosphatidylcholine (PC), a fundamental brain PL, is synthesised through the Kennedy pathway( Reference Kennedy and Weiss 47 ) from three precursors: choline, a pyrimidine, and, typically, a PUFA (either DHA or other PUFA). Phosphatidylethanolamine (PE) may be synthesised from a PUFA and a pyrimidine. These precursors act by enhancing the substrate saturation of enzymes that bring about the incorporation of the precursors in PC and phosphatidylethanolamine( Reference Wurtman, Ulus and Cansev 48 ). In accordance with this, it has been reported that synaptic proteins and PL are increased in gerbil brain by joint administration of uridine and DHA( Reference Wurtman, Ulus and Cansev 48 ). Furthermore, it was found that continuous supply of DHA, but not arachidonic acid (20 : 4n-6), may lead to an increase in brain phosphatide and synaptic protein levels according to animal models( Reference Cansev and Wurtman 49 ). Phosphatidylserine is also very important and abundant in the human brain and typically contains significant amounts of DHA( Reference Svennerholm 50 ). It is known that throughout childhood development DHA is accumulated within the brain PL, PC and phosphatidylethanolamine( Reference Martínez and Mougan 51 ).
Differently from EPA, DHA is not a source for eicosanoid synthesis, rather exerting influence directly and indirectly. DHA can also be converted to EPA by a retroconversion reaction, thereby leading to the formation of various eicosanoid metabolites( Reference Conquer and Holub 52 ). The DHA derivatives produced by oxidation reactions have also importance and are usually termed docosanoids( Reference Sawazaki, Salem and Kim 53 ). Such compounds bear resemblance to eicosanoids and are deemed as potential mediators of the biochemical processes in the central nervous system( Reference Sawazaki, Salem and Kim 53 ). DHA may also generate trans-4-hydroxy-2-hexenal (4-HHE) as a result of peroxidation. This oxidation product, 4-HHE, has been shown to be toxic to primary cultures of cerebral cortical neurons( Reference Long, Murphy and Leiphon 54 ). The formation of 4-HHE seems to follow an oxidation pathway different from that generating docosanoids. Hence, DHA may undergo different biochemical transformations as a function of the prevailing conditions and lead to distinct effects on the central nervous system.
Docosanoids include neuroprotectin D1 (NPD1), maresins, neuroprostanes (NeuroPs), and related 22-C derivatives( Reference Bazan, Molina and Gordon 55 ). The NeuroPs are structurally related to prostaglandins and constitute a large family of oxidised cyclopentanoid derivatives. NeuroPs are derived through a cascade of non-enzymic radical reactions from the non-enzymic peroxidation of DHA in neurons( Reference Porta, Pasi and Brunoldi 56 ). However, it has also been shown that lipoxygenase inhibitors block the synthesis of many docosanoids( Reference Bazan, Birkle and Reddy 57 ). Interestingly, it has been suggested that these DHA derivatives might be neuroprotective( Reference Bazan 58 ). The research into the role of NPD1 has brought forth evidence of such a neuroprotective effect( Reference Bazan 59 ).
NPD1 is attained from the selective oxygenation of DHA by the enzyme 15-lipoxygenase-1( Reference Calandria, Marcheselli and Mukherjee 60 ). NPD1 leads to homeostatic signalling in response to cellular and systemic imbalances( Reference Lukiw, Cui and Marcheselli 61 ). In particular, the positive regulatory actions of NPD1 together with DHA follow different interdependent mechanisms( Reference Brand, Schonfeld and Isharel 62 – Reference Rapoport, Rao and Igarashi 65 ). First, membrane properties encompassing lipid bilayer fluidity and membrane rafts are important for their biophysical characteristics. Another mechanism involves the recruitment and up-regulation of anti-apoptotic members of the Bcl-2 gene family. Moreover, the modulation of kinase-mediated Bcl-2 gene family phosphorylation is affected. The activation of inflammatory signalling mediators (for instance, the PG-synthesising arachidonic FA enzyme cyclo-oxygenase-2) is repressed. Finally, the expression of proapoptotic signalling is also repressed.
Different mechanisms for the DHA role as a protective agent against cognitive decline have been put forward. Namely, NPD1 may support brain cell survival and repair through neurotrophic, anti-apoptotic and anti-inflammatory signalling. Indeed, many of the effects of DHA on the neurological system may be related to signalling connections, thus leading to the study of the related signalolipidomics. However, the action of NPD1 as a possible modulating agent of transport mediated by ApoE and its effect on β-amyloid precursor protein (β-APP) processing, soluble amyloid precursor protein α fragment (sAPP-α) or amyloid-β peptide speciation, generation, and secretion during ageing, and in cytokine-, hypoxia- and oxidation-stressed human brain cell models of AD are not fully understood. DHA itself has been linked to these events( Reference Mukherjee, Chawla and Loayza 64 , Reference Cole and Frautschy 66 , Reference Kim, Akbar and Lau 67 ). It is still unsettled if, under those conditions, NPD1 is formed from DHA or if there are alternative mechanisms for DHA action( Reference Lukiw and Bazan 63 ).
However, there are aspects of the NPD1 action that need to be better understood, such as, the impact on the biophysics and kinetics of the membrane-embedded secretase-mediated cleavage mechanisms of β-APP( Reference Cole and Frautschy 66 , Reference Lukiw 68 ). Moreover, the effect of NPD1 on specific secretase activities is a still unexplored field, which deserves more attention, given its importance to the design of more effective and selective amyloid-β peptide-lowering agents( Reference Lukiw 68 , Reference Lukiw 69 ).
The Alzheimer’s Disease Cooperative Study AD study( Reference Quinn, Raman and Thomas 32 ) also suggests other biochemical interactions of DHA, given the sensitivity of ApoE4 allele-negative patients to DHA. It is known that ApoE can interact with various receptors in the brain, in neurons, astrocytes and in capillary endothelial cells at the blood–brain barrier( Reference Bu 70 , Reference Hauser, Narayanaswami and Ryan 71 ). ApoE4 is a lipid transporter, which may limit DHA transport in the brain. A comparison between old ApoE4 carriers with ApoE4 allele-negative individuals (carrying ApoE2 or ApoE3 alleles) points to a shorter DHA whole-body half-life in the former after an oral dose of [13C]DHA( Reference Chouinard-Watkins, Rioux-Perreault and Fortier 72 ). It has been reported that an accumulation of DHA in the blood is associated with lower concentrations in cerebral tissue of ApoE4 mice, taking ApoE2 animals as a reference( Reference Vandal, Alata and Tremblay 73 ). Such an inverse relationship between plasma and brain DHA contents suggests that plasma levels( Reference Plourde, Vohl and Vandal 74 ) may reflect defective distribution in the brain rather than being a good correlate of brain DHA content. So, it seems that ApoE4 leads to less DHA being transported into the brain, thereby causing a deleterious effect in AD( Reference Salem, Vandal and Calon 21 ).
A further mechanism relating DHA dietary intake and cognitive function ageing may involve the role of DHA in inflammatory processes. Indeed, DHA and EPA are deemed to display some anti-inflammatory properties( Reference Bradbury 75 , Reference Calder 76 ), thereby offsetting the pro-inflammatory effects of n-6 PUFA( Reference Calder 76 ). For diseases having a recognised central role of inflammation to the pathology such as asthma or rheumatoid arthritis, DHA supplementation in the diet may be protective. The DHA-derived docosanoids are potent endogenous anti-inflammatory and pro-resolving chemical mediators( Reference Serhan 77 ). They may reduce chronic inflammation by attenuating NF-kB, thereby modulating the expression of pro-inflammatory cytokines. On the other hand, abundant evidence indicates that inflammatory processes are active in AD( Reference Freund-Levi, Hjorth and Lindberg 78 ). Epidemiological studies indicate a lower prevalence of AD in individuals treated with non-steroidal anti-inflammatory drugs, but clinical trials have not yielded strong effects( Reference Meinert and Breitner 79 ). It is known that AD is related to the activation of microglia by different factors, including β-APP and pro-inflammatory cytokines( Reference Patel, Paris and Mathura 80 ). Microglia increase the levels of some cytokines, such as IL-6, and TNF-α, which may generate deviations from the normal neuronal function( Reference Schultzberg, Lindberg and Forslin 81 ).
Besides, DHA incorporation into the cell membranes modulates the efficiency of numerous membrane transporters and enzymes( Reference Srinivasarao, Narayanareddy and Vajreswari 82 ). The incorporation of DHA into cell membranes is of great importance, since many essential cellular processes take place in and on membranes( Reference Klose, Surma and Simons 83 ). These processes are affected by the biochemical and biophysical properties of organelle membranes. Precisely, the lipid composition of these membranes influences the membrane properties, which, in turn, decisively exert an effect upon the activity of membrane-embedded proteins( Reference Lee 84 ). For instance, membrane thickness can affect the location of proteins.
DHA may also affect directly the physical properties of membranes, which depend on PL that are known to have a large importance in the neural membranes. For instance, PL, such as glycerophospholipids and sphingolipids, and sterols are prominent lipid classes in the membranes, but there is a large diversity of other minor lipid components( Reference Shevchenko and Simons 85 ). The physical properties of membranes are affected both by the head groups and the hydrocarbon chains of lipid molecules. These effects can be tremendous not only on the properties, but also on the processes occurring within the membranes, even with subtle changes in lipid composition( Reference Klose, Surma and Simons 83 ). For instance, while a hypothetical bilayer of PC with two chains of a SFA such as stearic acid (18 : 0) displays a packed ordered state without any diffusion of lipid substances, a bilayer of PC with two DHA chains exhibits a more disordered state with freely moving lipid molecules( Reference Kahya, Scherfeld and Bacia 86 , Reference Silvius 87 ). Moreover, longer FA chains and a higher content of sphingolipids and sterols in the membrane correlate with an enhanced thickness( Reference Surma, Klose and Klemm 88 ). It has also been observed that asymmetric distribution of glycerophospholipids and sphingolipids between the two leaflets of the neural membrane may lead to dynamic lipid substructures( Reference Glomset 46 ). Therefore, the connections between DHA and the membrane physical properties are another important research field deserving further scientific studies.
Future research on the mechanistic aspects connecting DHA and AD as well as other cognitive ageing disorders should also identify and quantify relevant biomarkers in the plasma and cerebrospinal fluid, bridging the gap between docosanoids, cytokines and neuronal cell changes.
DHA and the cognitive function
The effects of DHA on cognitive ageing need an understanding of the multiple connections between DHA and the highest degrees of brain activity. Several studies have been conducted regarding this subject (Table 3). A deficient level of DHA is related with changes in the operation of cognitive function, namely, in ageing, hyperactivity, AD, schizophrenia and peroxisomal diseases( Reference Bazan, Molina and Gordon 55 ). Conversely, higher dietary intake of DHA is linked to better brain health( Reference Burri 89 ). Indeed, DHA is enriched in synaptic membranes, being able to change their fluidity as well as neurotransmitter and receptor densities. These mechanisms whereby DHA affects neural cells have already been described in previous section, but more studies on their details and the way that DHA positively affects cognitive function are warranted. There are several studies of a medical nature pointing to the positive effect of DHA on cognitive function( Reference Burri 89 ), but the full understanding of the underlying biochemistry remains elusive.
Table 3 Summary of the main studies concerning DHA and cognitive function

ALA, α-linolenic acid.
Many studies relate to human cognitive function evolution as a result of ageing. For instance, a study on the effects of a 90 d DHA supplementation (252 mg/d) on cognitive function in a healthy ageing population did not find any significant impact( Reference Stough, Downey and Silber 90 ). Besides, it has been argued that there is greater evidence for DHA playing a preventive rather than curative role in dementia( Reference Cunnane, Plourde and Pifferi 27 ). This role may be more important in unhealthy populations, for instance, in patients with type 2 diabetes( Reference Zhao, Gillam and Taylor 91 ). Namely, it is not clear if an adequate brain DHA level can be kept in obesity and insulin-resistant states. Indeed, it is quite possible that the DHA level becomes inadequate, given evidence of greater cognitive decline in individuals with insulin resistance( Reference Young, Mainous and Carnemolla 92 ). Moreover, it has been reported( Reference Hashimoto, Katakura and Tanabe 93 ) that reference memory-related learning ability is positively correlated with DHA-derived docosanoids in aged rats. The same study did not find a significant correlation for EPA-derived mediators. Moreover, dietary DHA improves the learning-related spatial memory of DHA-deficient rats( Reference Hashimoto, Tanabe and Fujii 94 , Reference Mills, Hadley and Bailes 95 ).
There is a lack of robust evidence to evaluate the effect of DHA in diet on the cognitive performance of young healthy adults. Some of the trials that have been done seem to present experimental design shortcomings. For instance, a placebo control is absent( Reference Fontani, Corradeschi and Felici 96 ), sample size is small( Reference Antypa, Van der Does and Smelt 97 ) and duration is too short( Reference Jackson, Deary and Reay 98 , Reference Rogers, Appleton and Kessler 99 ). On the other hand, a cross-sectional study on adults aged between 30 and 70 years old showed a positive association between DHA blood levels and scores on cognitive performance tests( Reference Muldoon, Ryan and Sheu 11 ). Against this backdrop, a recent work involving RCT has shown that DHA supplementation has improved both memory and reaction time in healthy young adults( Reference Stonehouse, Conlon and Podd 9 ). It should be remarked that the habitual diet of these young adults (age range 18–45 years) was low in DHA. Moreover, response was modulated by sex – whereas DHA improved episodic memory in women, it improved reaction times of working memory in men( Reference Stonehouse, Conlon and Podd 9 ). Another recent study provided compelling initial evidence that dietary factors affect the connection between physical activity and cognitive performance( Reference Leckie, Manuck and Bhattacharjee 100 ). In particular, high levels of DHA relative to arachidonic acid reduced the negative effects of lower physical activity on performance. The results of these two studies may be related to the fact that DHA accumulates in areas of the brain involved in memory and attention such as the cerebral cortex and hippocampus( Reference Chung, Chen and Su 101 , Reference Gamoh, Hashimoto and Sugioka 102 ). Nevertheless, further observational studies and RCT are warranted in order to achieve a higher degree of certainty and a deeper understanding of the connections.
Dietary sources of DHA, bioaccessibility and bioavailability
The large importance of DHA makes this an essential FA in human nutrition. Diets should be formulated in order to ensure an adequate level of DHA supply. The main source of DHA is seafood, particularly marine fish and shellfish( Reference Ackman, Ratnayake and Olsson 103 ). DHA is found in the flesh of both lean and oily fish, with much greater amounts in the latter, and in the liver of some lean fish species, such as cod. There is also fish oil prepared from these raw materials rich in DHA( Reference Calder 76 ). There are also non-marine sources of DHA. However, DHA contents are only comparable with lean fish in the case of some meat-processing by-products and especially enriched foods. An overview of the DHA content in different sources and their characteristics is presented in Tables 2 and 4. Since meat, cereals and milk are more important in the Western diet, DHA intake is low( Reference Howe, Meyer and Record 104 ). Indeed, for a total of approximately 100 mg DHA/d, fish and seafood products are the largest contributor with 69·9 mg/d, followed by meat products with 19·6 mg/d, and egg products with 5·1 mg/d.
Table 4 Non-marine DHA dietary sources and their main characteristics, advantages and drawbacks

* Values from Fonollá et al. ( Reference Fonollá, López-Huertas and Machado 133 ) and Klop et al. ( Reference Klop, Hatew and Bannink 134 ).
† Values from Lemahieu et al. ( Reference Lemahieu, Bruneel and Ryckebosch 135 ).
‡ Values from Woods & Fearon( Reference Woods and Fearon 136 ) and Zotte & Szendrö( Reference Zotte and Szendrö 137 ).
§ Values from Sobol et al. ( Reference Sobol, Skiba and Raj 138 ).
DHA is present primarily as TAG and, to a lesser extent, as NEFA in fish and derived unrefined raw oils( Reference Schuchardt and Hahn 105 ). In krill oil, a third fraction is found, since a substantial percentage of n-3 PUFA (and DHA) is bound in PL( Reference Schuchardt and Hahn 105 ). Pharmaceutical-grade, highly concentrated fish oil supplements with DHA bound in ethyl ester, are also available( Reference Calder 76 ).
Oily fish, such as herring, salmon and sardine, are the richest sources of DHA( Reference Harris, Kris-Etherton and Harris 106 ). According to these authors, of thirty-seven commonly consumed types of fish products, DHA is the main n-3 PUFA, being on average 65 % of total n-3 PUFA( Reference Afonso, Lourenço and Cardoso 107 ). It should be remarked that DHA content in fish usually varies with the overall n-3 PUFA content. Three main classes of fish products may be differentiated on the basis of DHA content: relatively poor DHA sources (black scabbardfish, catfish, hake, megrim, tilapia); moderately rich DHA sources (halibut, pollock); and very rich DHA sources (herring, mackerel, salmon, sardine), corresponding to the approximate ranges <300, 300–500, and >500 mg/100 g, respectively( Reference Harris, Kris-Etherton and Harris 106 – Reference Nunes, Bandarra and Oliveira 111 ).
For a more detailed presentation of DHA concentrations in different marine sources, Table 2 based on the Portuguese Institute for the Sea and Atmosphere (IPMA) extensive database( Reference Nunes, Bandarra and Oliveira 111 , Reference Bandarra, Calhau and Oliveira 112 ) and different papers( Reference Peinado, Girón and Koutsidis 113 ) can be consulted. The six highest DHA contents are found in the European eel, chub mackerel, Atlantic salmon, Atlantic mackerel, gilthead seabream (wild) and sardine, all exceeding 1000 mg/100 g( Reference Nunes, Bandarra and Oliveira 111 , Reference Bandarra, Calhau and Oliveira 112 ).
The American Heart Association’s recommended daily intake (RDI) is 500 mg EPA + DHA for individuals without CHD( Reference Kris-Etherton, Harris and Appel 114 ). The European Food Safety Agency has advised 250 mg of EPA + DHA( 115 ) and reference values for the EPA+DHA RDI are typically in the 250–500 mg range( 116 ). Specifically for DHA, an RDI of 250 mg has been put forward by ANSES (Agence Nationale de Sécurité Sanitaire de l’Alimentation, de l’Environnement et du Travail)( 117 ). A single weekly meal of 150 g of chub mackerel, Atlantic salmon or sardine may be more than enough to meet this DHA RDI (250 mg/d). For seafood moderately rich in DHA, the consumption of two to three weekly meals of 150 g may also be enough.
The level of DHA in a portion of food that is eaten may be quite different from the bioaccessible level, that is, the DHA concentration that is released from the food matrix into the intestinal lumen after digestion and is available for absorption( Reference Cardoso, Afonso and Lourenço 118 , Reference Paustenbach 119 ). On the other hand, bioavailability is usually defined as the fraction of an oral dose of a substance that reaches the systemic circulation( Reference Schumann, Classen and Hages 120 ). The bioaccessible content is always equal or higher than the bioavailable content( Reference Cardoso, Afonso and Lourenço 118 ). Bioaccessibility is usually determined by in vitro simulations of human digestion( Reference Cardoso, Afonso and Lourenço 118 , Reference Versantvoort, Oomen and Van de Kamp 121 ). For bioavailability, according to the definition given above, cell lines and transwell assays are used for mimicking the intestinal lining barrier( Reference Minoo, Moyer and Jass 122 ) and cell cultures simulating the relevant liver tissues may also be used( Reference LeCluyse, Witek and Andersen 123 ). Bioaccessibility and, as a consequence, bioavailability of DHA may depend on the chemical binding form (DHA bound in ethyl ester, TAG or PL) (Fig. 1), matrix effects (fat and other components content in food), and, in the case of DHA in supplements, galenic form (microencapsulation, emulsification, etc.)( Reference Schuchardt and Hahn 105 ).
DHA biosynthesis routes
Besides dietary DHA and the bioaccessibility/bioavailability issues, DHA may be biosynthesised in the human body. However, for healthy and non-vegetarian humans, despite the availability of the necessary enzymes, there is extremely limited synthesis of DHA in adults( Reference Pawlosky, Hibbeln and Novotny 124 , Reference Plourde and Cunnane 125 ). Unless induced by several years of a vegetarian diet, the human enzymic machinery is very inefficient in converting, for instance, ALA to EPA and DHA. Even with a diet deficient in DHA, the brain cells’ ability to synthesise DHA from ALA is very low( Reference Igarashi, DeMar and Ma 126 ). One study indicates a very low share of plasma ALA, < 0·2 %, deployed to the synthesis of DHA via EPA( Reference Pawlosky, Hibbeln and Novotny 124 ). Indeed, it has been claimed an extremely low level of conversion of the precursor ALA to EPA, < 5 %( Reference Brenna 127 ), and to DHA, < 0·05 %( Reference Burdge, Finnegan and Minihane 128 ). Several enzymes are required to elongate and desaturate ALA or other shorter and less unsaturated n-3 PUFA into DHA. Research has found evidence suggesting that DHA formation may be regulated independently of other FA in the pathway and that DHA binding to PPARα suppresses transcription of the ∆-6 desaturase gene, thereby down-regulating conversion of ALA to DHA( Reference Tang, Cho and Nakamura 129 ). Indeed, it should be noted that the rate-limiting step in DHA synthesis is precisely the desaturation of ALA by ∆-6 desaturase. An overview of the possible routes for attaining DHA in the human organism, taking into account enzyme action, conversion rates, genetic factors, and dependence on the starting n-3 PUFA, is presented in Table 5.
Table 5 Possibilities for attaining DHA from different precursors in the human organism, conversion rates associated with DHA synthesis, genetic factors and performance in producing DHA for each precursor

ALA, α-linolenic acid; SDA, stearidonic acid.
Conclusions
DHA is mainly found in seafood, being rich sources of DHA such as marine fish and shellfish. Oily fish such as herring, salmon, sardine and tuna provide the highest amount of DHA per meal. DHA intake may be associated with several health endpoints ranging from inflammatory processes, asthma and rheumatoid arthritis to CVD and diabetes mellitus as well as to depression and cancer. Particularly, DHA has an important role in the nervous system, which is highlighted by its prominence in neural tissues. DHA may lead to the formation of docosanoids such as NeuroPs or NPD1. Namely, the action of NPD1 in the central nervous system is influential in different ways. It is known that NPD1 leads to homeostatic signalling in response to cellular and systemic imbalances. Nevertheless, much needs to be known about the mechanisms and roles of NPD1. For instance, NPD1 as a possible modulating agent of transport mediated by ApoE and its effect on β-APP processing is not fully understood. In spite of this, there seems to be some protection against cognitive decline with ageing and even improved memory and reaction time in healthy young adults. Indeed, for ageing-related MCI, some studies suggest that DHA may improve cognitive abilities. Nonetheless, for healthy subjects or MCI and AD patients, the evidence is still not convincing. In this context, it is worthwhile noting that for ApoE4 allele-negative AD patients, DHA produced a benefit in the cognitive score. Future studies should take the DHA bioavailability issue into account in order to achieve better results. On the other hand, research should try to separate the role of DHA and of EPA through studies using DHA only instead of fish oil rich also in EPA. Moreover, DHA in each main lipid class should be quantified instead of global DHA. Finally, future RCT and observational studies should always take into account the genetic traits of the population, since some effects may only be detected in subgroups with specific alleles.
Acknowledgements
C. A. and C. C. acknowledge the individual Post Doctoral Grant (no. SFRH/BPD/64951/2009 and no. SFRH/BPD/102689/2014, respectively) funded by the ‘Fundação para a Ciência e a Tecnologia’ (FCT).
The planning as well as the final reading and reviewing of the paper was carried out by all three authors. C. C. wrote three sections, C. A. wrote another three sections, and N. M. B. wrote the last section and coordinated.
There are no conflicts of interest.