1. Introduction
Obtaining paleoatmospheric information on both regional and global scales is a key objective in ice-core research. The basis for such reconstructions is the fact that concentrations of chemical (and gaseous) species found in snow and ice are determined in part by the atmospheric concentrations of those species, and additionally by depositional and post-depositional processes. For certain soluble species (i.e. nonvolatile sea-salt and erustally derived ions), reconstruction of relative changes in paleoatmospheric concentrations is possible since post-depositional changes are negligible. For other species, such as NO3 − and H2O2, both depositional and post-depositional processes in snow and ice can alter preserved concentrations of certain species, making interpretation to past atmospheric concentrations more difficult (Reference Dibb, E. W. and BalesDibb, 1996). Post-depositional processes in most cases do not significantly alter long-term average concentrations, but can affect short-term (i.e. sub-annual scale) fluctuations, as well as the amplitude and timing of chemical signal seasonality (Reference Wolff, Wolff and BalesWolff, 1996).
An example of such a process is the recently observed post-depositional migration of methane sulfonic acid (MSA), an oxidation product of marine biogenically produced dimethyl sulfide (DMS) (Reference Saltzman and DelmasSaltzman, 1995). A shift from summer (near the surface) to apparent winter (below ~5—10 m depth) MSA deposition has been observed in Antarctic ice cores from Dolleman Island (Reference Mulvaney and WolffMulvaney and others, 1992), Byrd Station (Reference Langway, Osada, Clausen, Hammer, Shoji and MitaniLangway and others, 1994), the Filchner-Ronne Ice Shelf (FRIS; Reference Minikin, Wagenbach, Graf and KipfstuhlMinikin and others, 1994) and Berkner Island (Wagcnbach and others, 1994). Because a change in the seasonal production and deposition of MSA is unlikely (Mulvaney and others, 1992), migration of MSA relative to excess (xs) SO4
2- (which continues to peak during maxima in the Dolleman Island core, and is therefore assumed 10 remain in place after summer deposition) is thought to have occurred. Interpretation of MSA/xsSO4
2- molar ratios (JR) at these sites, especially where high-resolution sampling is performed (>5 samples a−1), can be seriously complicated by post-depositional migration of MSA (Mulvaney and others, 1992). This phenomenon, however, has not yet been documented at enough sites to provide a clear spatial understanding of where and under what conditions it occurs.
Like MSA, post-depositional processes are known to affect NO3 − concentrations in snow and ice. There is compelling evidence for HNO3 loss in surface snow at low-accumulation-rate sites (Mayewski and Legrand, 1990; Dibb and Whitlow, 1996), possibly through photochemical degradation or degassing (Reference Neubauer and HeumannNeubauer and Heumann, 1988). Diffusive smoothing of seasonal NO3 − patterns in firn has also been noted ( Wolff, 1996). Further, NO3 depletion has been observed in ice layers impacted by deposition ofvolça-nically derived acids (mainly H2SO4) (Reference Laj, Palais, Gardner and SigurdssonLaj and others, 1993; Reference Yang, Mayewski, Zielinski, Twickler and TaylorYang and others. 1996;. While it is possible that changes in the atmospheric nitrogen cycle are responsible for decreased NO3 − deposition during volcanic eruptions, an alternate explanation involves post-depositional migration of NO3 − from the highly acidic volcanic-ice layers (Wolff, 1996). Clearly, the behavior of NO3 − in acidic firn and ice layers needs to be understood so that proper interpretations of glaciochemical data can be made.
Chemical species derived from sea-salt aerosols (Na+, CI− , Mg2+, K+, Ca2+), which can be used as proxy indicators of past atmospheric circulation strength (e.g. Mayewski and others, 1994), are considered to be conservative, non-reactive, and therefore fixed in place after deposition in most locations. Wolff (1996), however, demonstrated that Mg2+ concentrations peak on either side of Na+ winter maxima in the Dolleman Island ice core, causing disruption in standard Mg2+/Na+ marine ratios. Although the migration of Mg2+ in the Dolleman Island core was confined with in the same annual layer, paleoatmospheric circulation reconstructions based on multivariate statistical techniques (e.g. Mayewski and others, 1994) could potentially be affected by such migration. The problem may be enhanced in ice-age ice (in both Antarctica and Greenland), where sca-salt concentrations can be ~30-40 times higher than modern values (Reference Palais and LegrandPalais and Legrand, 1985). Dolleman Island is the only location so far where this phenomenon has been observed; therefore a more thorough investigation of the problem is needed to determine under what conditions it occurs.
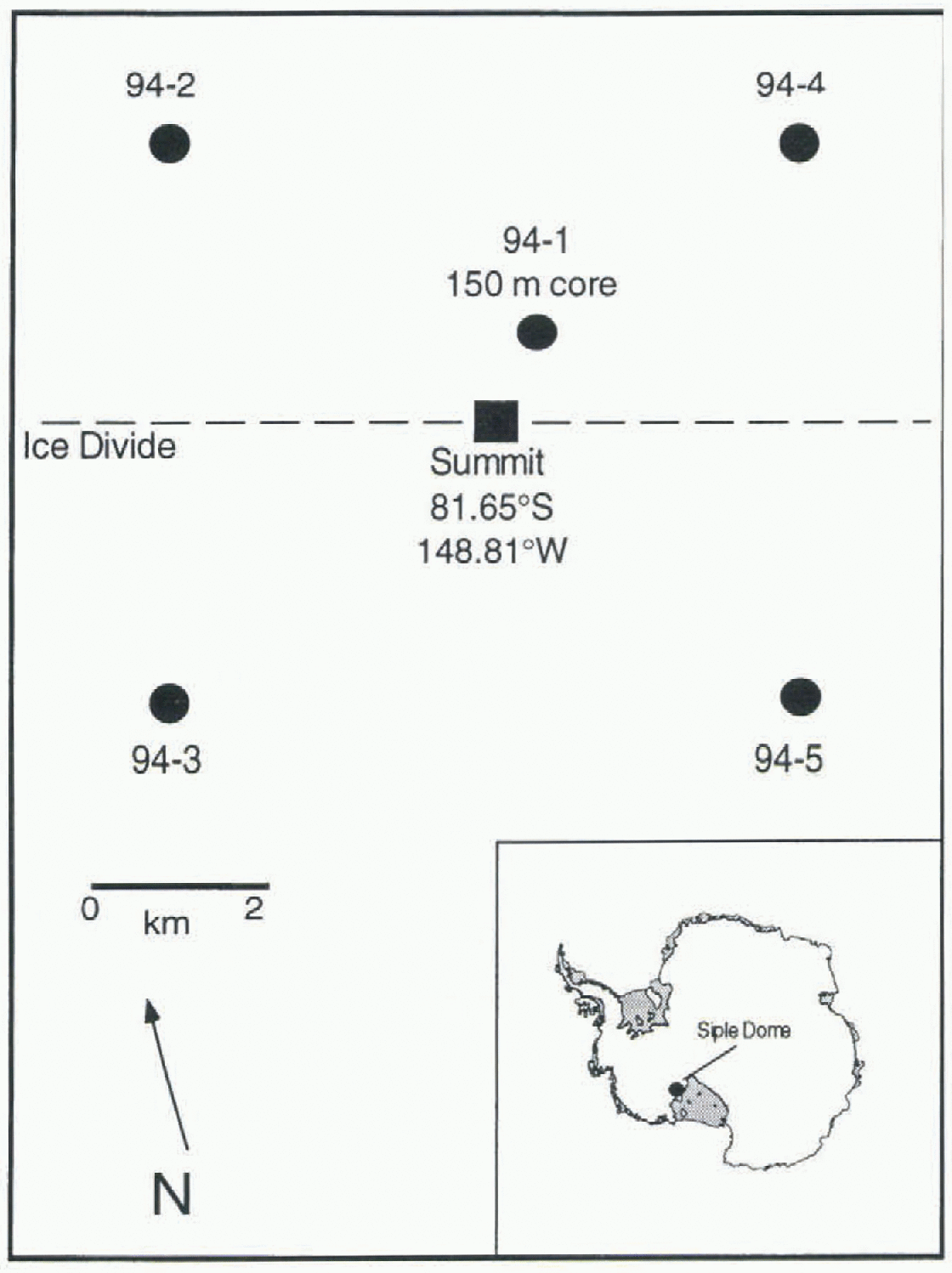
Fig. 1. Location map of 1994 sampling sites cm Siple Dome, Antarctica. Pit 94-1 was sampled to 4 m depth, while pits 94-2, -3, -4 and -5 were sampled to 2 in depth. Elevation of the Siple Dome summit is 621 m.
In this paper, we present results from a LSiple Dome, Antarctica (81.65° S, 148.81°W; Fig. 1), ice-core glaciochemical record and investigate migration of the soluble chemical species described above. Compared to other sites where similar ion-migration phenomena have been observed, Siple Dome is unique in its high sea-salt and marine biogenic acid concentrations and relatively low accumulation rate (13.3 cm ice a−1; Kreutz and others, 1997). One result of these physical characteristics is a seasonal alternation between deposition of more acidic summer layers (H2SO4, HNO3, MSA) and less acidic winter layers (sea salt). Because both sea-salt concentrations and accumulation rale have been suggested as possible controls on species migration (WohT, 1996), Siple Dome provides an ideal location to investigate these processes.
2. Methods
During the 1994-95 field season, a 150 m core was drilled ~3 km north-northeast of the summit of Siple Dome (10 m temperature -25°C; Mayewski and others, 1995; Fig. 1). in addition, five snow pits were sampled in November 1994: a4 m pit at the drill-site, and 2 m pits at each corner of a 10 km × 10 km surveyed grid centered at the drill-site (Fig. 1). Snow-pit sampling and ice-corc collection was performed by workers using non-particulating suits, polyethylene gloves, and particle masks. A 2cm interval was used in snow-pit sampling.
Core samples were processed in a cold room at temperatures not exceeding -12°C, by individuals wearing pre-cleaned polyethylene gloves and particle masks. All tools and containers used were thoroughly pre-cleaned using ultra-pure water. The upper 2 m of core were not processed, due to poor core quality. As there is reasonable agreement between chemistry data from 2-4 m depth in the core and the 4 m core-site snow pit, the upper 2 m of pit 94-1 are used to create a continuous 24 m core record. Blanks of frozen ultra-pure water prepared at frequent intervals indicate that sample containers and techniques were free of contamination. Analysis of major cations (Na+, K+, NH,+, Mg2+, Ca2+), anions (Cl−1, NO3 −, SO4 2-) and MSA in core and snow-pit samples was performed at the University of New Hampshire using Dioncx 4000 series instruments. Cations were analyzed via suppressed chromatography with a Dionex CS12 column, 0.125 μl loop and 20 mM MSA cluent. Anions were analyzed with a Dionex ASH column, 75μ1 loop and 6mM NaOH eluent. MSA measurements were made with an ASII column, 1.5 ml loop and 6 mM NaOH eluent.
Dating of the core and snow-pit samples was done using well-preserved annual chemical signals in both discrete and continuous melter sampling (notably xsSO4 2- (see below) and CI−), beta radioactivity profiles, snow-pit and core stratigraphy, and volcanic marker horizons (Mayewski and others, 1995; Kreutz and others, 1997). Based on the depth/ age scale developed for the core, the 1150 year average accumulation rate is ~13.3 cm ice a−1. The upper 24 m of the core was sampled at 2 cm intervals to provide a high-resolution (at least 8 samples a−1) glaciochemical record of the past 110 years.
Calculation of sea-salt (ss) and non-sea-salt (xs) portions of chemical species in Siple Dome snow-pit and core samples is based on bulk seawater ratios according to the formula (Reference Delmas, Kirchner, Palais and PetitDelmas, 1992):

Na+ is used as the reference species, as it was found to be the most conservative sea-salt element at Siple Dome (Kreutz and others, 1997). in the case of SO4 2- , SO4 2- is the sum of (1) acidic sulfate and (2) the deficit (or excess) of SO4 in sea-salt aerosol (Mulvaney and Wolff, 1994). Using a k value which reflects bulk seawater [SO4 2-]/[Na+] mass ratio (0.252), calculations for winter snow and firn samples often lead to negative xsSO4 2- estimates at Siple Dome and other Antarctic coastal sites (Reference Wagenbach, Wolff and BalesWagenbach and others, 1988; Reference GjessingGjessing, 1989; Mulvaney and others, 1992; Minikin and others, 1994). The deficit suggests that fractionation must be occurring between bulk seawater and sea-salt aerosol during parts of the year, but flow such fractionation occurs is unclear. One possibility (Reference DucrozDucroz, 1996) is precipitation of Na2SO4 in seawater below 8°C, resulting in a brine solution that is depleted in SO4 2- (the relative depletion of Na+ would be negligible since it is present in much higher concentrations). To correct for this problem, winter layers were separated for each snow pit and the upper 6 m of core. SO4 2- and Na were plotted to calculate the slope (k). Our results (table 1) are similar to those obtained at other Antarctic sites. Therefore, we have used a k value of 0.1 to calculate Siple Dome snow-pit and ice-core xsSO4 2- values. Because there is no evidence for SO4 2- fractionation during summer months, and our calculation is not seasonally dependent, summer xsSO4 2- values may be overestimated in some-cases.
Table 1. Estimated sulfate to sodium mass ratio (k) in sea-salt aerosol at Siple Dome

3. Results and Discussion
3.1. MSA
MSA and xsSO4
2- concentrations from the upper 2 m of the Siple Dome core are presented in Figure 2. The two species are in phase through the upper 1.5m (approximately 3.5 years). in addition, the last 3 years of MSA and xsSO4
2- maxima correspond with summer peaks. These findings are consistent with aerosol measurements at Neumayer Station, which are well correlated with the annual DMS cycle measured at the sub-Antarctic Macquairie Island (Reference Gillet, Ayers, Ivery, Gras, Rostolli and AngelettiGillet and others, 1993), suggesting that the three are directly related (Wagenbach, 1996). Likewise, xsSO4
2- and MSA concentrations in surface snow from coastal locations (KRIS (Minikin and others, 1994)) and inland locations (South Pole (Whitlow and others, 1992); Dronning Maud Land (Reference OsadaOsada, 1991)) demonstrate strong seasonal signals that both [leak in the summer. The xsSO4
2- /MSA relationship observed in the upper 1.5 m of the core (coeval existence in the first 3.5 years; not in depth due to small differences in accumulation rate between the north and south sides of the Siple Dome ice divide) is consistent in all Siplc Dome snow pits collected in 1994 (Fig. 1).
Below 1.5 m in the core, MSA peaks occur slightly before (shallower than) xsSO4 2- peaks (Fig. 2). As previously discussed, we assume that this represents post-depositional migration of MSA and not a significant shift in deposition liming. This pattern (the first sign of MSA migration after the first 3 years) is spatially consistent over the 10 km x 10km grid on which six snow pits were collected (Fig. 1). Apparent initial migration of MSA at Siple Dome after 3 years is earlier than ai Dolleman Island (4.4 m, or ~5 years; Mulvaney and others, 1992), Byrd Station (4.6 m, or ~13 years; Langway and others, 199 11, Berkner Island (3.5 m, or ~12 years; Wagenbach and others, 1994) and the FRIS (1.8 m, or ~8 years; Minikin and others, 1994).
Deeper in the core, MSA and xsSO4 2- peaks are clearly out of phase (Fig. 3). Specifically, below 2 m depth (selected 2 m sections of the core are presented in Figure 3), there is no place where MSA and xsSO4 2- are in phase. This situation is unlike Dolleman Island and Byrd Station cores, which contain a transition zone where the MSA/xsSOf relationship is both in and out of phase. At Siple Dome. MSA migration appears to occur early and without a transition zone. At least one MSA peak exists between each xsSO4 2- peak, suggesting that the distance of MSA migration must be limited to one season.

Fig. 2. Glaciochemical (μeql−1) and isotopic (per mil (‰)) concentrations in samples (2 cm sum pie interval) from the upper 2 m of Siple Dome snow. Approximately 5.5 years of snow deposition are contained in the 2 m section. Dates given above each plot represent 1 January of that year.

Fig. 3. xsSO4 2- and MSA concentrations (μeql−1) in samples (2 cm sample interval) from four sections of the Siple Dome ice core. Corresponding dates are given above each plot.
Composite mean annual chemical cycles from different depths in the core (Fig. 4) also indicate MSA migration. Values of xsSO4 2- consistently peak during the austral summer in three different core sections. MSA values also indicate peak values during summer months in upper core samples. in the two lower sections, however, MSA values clearly have shifted to winter peaks. The apparent decrease in amplitude may be a function of fewer samples per year deeper in the core, and also the possible role of the prolonged early 1990s El Niño Southern Oscillation (ENSO) event (Trenberth and Hoar, 1996) in producing very high MSA values in surface snow (~1991-94). Although the reduction in amplitude would suggest diffusive smoothing of MSA, peak shapes appear to remain sharp and well defined throughout the upper 24 m (Fig. 3), and therefore the amount of diffusive smoothing must be limited.
3.2. NO3 −
NO3 − concentrations from the upper 2 m of the Siple Dome core are presented in Figure 2. Peak values of NO3 − occur on the rising limb of NO3 − cycles and slightly earlier than xsSO4 2- peaks (Fig. 2), suggesting that xsSO4 2- deposition occurs during late spring when the polar vortex initially breaks down. This is consistent with other observations of the annual NO3 − cycle in Antarctic surface snow and air Mayewski and Legrand, 1990; Reference Savoie, Prospero, Larsrn and SaltzmanSavoie and others, 1992; Whitlow and others, 1992; Mulvaney and Wolff, 1993; Minikin and others, 1994; Osada, 1994; Wageribach, 1996). This pattern (no apparent movement of NO3 − relative to xsSO4 2- in the upper 2 m) is spatially consistent over the 10 km x 10 km grid on which five snow pits were collected. Unlike very low-accumulation-ratc sites (i.e. South Pole, Vostok), Siplc Dome gives no indication of significant NO3 − enrichment in near-surface snow due to post-depositional degassing of NO3 − (Fig. 2).
In Figure 5, NO3 − concentrations are plotted vs xsSO4 2- (summer indicator) for selected core sections in Figure 5. NO3 − concentrations consistently peak slightly earlier than xsSO4 2- to a depth of 8.5 m. in the 8.5-10 m section, the phase NO3 − /xsSO4 2- relationship becomes confused. Below 10 m (10-12 m), it appears that NO3 − has migrated into the winter layer in some places, but is still in phase with xsSO4 2 in other places. By 14-16 m, NO3 − has clearly moved into winter layers during every annual cycle. Likewise, in the 18-20 m section, NO3 − and xsSO4 2- are anticorrelated. in each core section, there is at least one NO3 − peak for every xsSO4 2- peak, suggesting that the distance of NO3 − migration must be limited to one season.

Fig. 4. Alean annual cycles of Na+, Mg2+, xsSO4 2-, MSA, and NO3 − in the upper 24 m of the Siple Dome core. Concentration values (μeql−1) are given as deviations from the 110 year mean of each species. The thick line corresponds to the near-surface depth interval, which overlaps snow-pit samples and the time interval (1988-94) of the prolonged early 1990s ENSO event, and contains the interval where MSA migration is first noted. The thin line represents the interval where NO3 − migration is first noted, and the thin dashed line is the interval where pronounced Mg migration is observed.

Fig. 5. Fig. 5. xsSO4 2- and NO3 − concentrations (μeql−1) in samples (2 cm sample interval) from jour sections of the Siple Dome ice core. Corresponding dates are given above each plot.
Composite mean annual NO3 − values (Fig. 4) indicate summer peaks in upper core samples. in 1960-68 samples, there is still evidence of summer NO3 − peaks, although the signal is much less pronounced than during 1988-94. in 1910-22 samples, NO3 − values have shifted to winter peaks. There appears to be some loss of signal amplitude with increasing depth, which may be related to sampling and/or diffusive smoothing. The amount of diffusive smoothing occurring must be limited, as NCV peaks retain a sharp character throughout the entire 24 m of detailed core data (Fig. 5).
3.3 Mg2+
Mg2+ concentrations in the upper 2 m of the Siple Dome ice core are presented in Figure 2. Mg2+ and Na+ are well correlated (and in marine ratio), suggesting that Mg2+ at Siple Dome is mainly derived from sea-salt aerosol (Fig. 3). Peaks in Mg2+ and Na− generally occur during δD minima, indi-cating that most of the sea salt is deposited during winter. Aerosol measurements at Antarctic coastal stations (Neumayer (Wagcnbach, 1996); Mawson (Savoie and others, 1992)) and inland stations (South Pole (Tunecí and others, 1989)), as well as surface snow measurements (Whitlow and others, 1992; Osada, 1994), also reveal a seasonal cycle in sea salt which peaks in the winter season. However, short-term sea-salt increases have also been observed throughout the year at Mawson and Neumayer stations, and are linked to local storm activity (Wagenbach, 1996). A similar situation is observed at Siple Dome, where input of sea salt occasionally occurs during summer (i.e. 1992, 1993). Because the sea-salt cycle is out of phase with seasonal sea-ice fluctuations, transport of sea-salt aerosols during winter is most likely by rapid long-range advection from large open-water areas as well as from local polynias opened by heavy storm winds (Wagenbach, 1996). The correlation between Na+ and Mg is consistent in the top 2 m of other Siplc Dome snow pits (Fig. 1).
The first indication of Mg2+ migration (relative to Na+) occurs at 9.65 m depth in the core, and is continued throughout the remaining 11 m of detailed data (Fig. 6). As in the Dolleman Island core (Wolff, 1996), migration of Mg2+ is characterized by Mg2+ peaks forming on either side of an Na peak. The first indication of Mg2+ migration at Dolleman Island occurs below about 10 m (Wolff, 1996). Therefore, comparison of the problem in the two cores suggests that Mg2+ migration begins at a similar depth, but at different times (~33 years at Siple Dome; ~13 years at Dolleman Island).The lowest Na+ concentration at which Mg2+ migration occurs at Siple Dome appears to be

Fig. 6. Na+ and Mg2+ concentrations (μeql−1) in samples (2 cm sample interval) from four sections of the Siple Dome ice core. Corresponding dales are given above each plot.
~μeql−1 Nearly every Na+ peak above 15μeql−1 has Mg2+ peaks formed on either side. Based on limited data (39.6-42.5 m), a similar Na+ threshold exists at Dolleman Island (Wolff, 1996).
Comparison of mean annual Mg2+ and Na+ cycles (Fig. 4) indicates coeval winter peaks in upper core samples. in 1960-68 samples, there is still an identifiable winter Mg + peak, but it is weaker than in the upper part of the core. in 1910-22 samples, Mg2+ clearly peaks in the summer. This 2 m section of core (18-20 m; Fig. 6) contains a number of Mg2+ peaks that are directly out of phase with Na+. There is no apparent reduction in Mg2+ peak amplitude, and Mg2+ peaks retain a sharp character throughout all 24 m of high-resolution samples.
3.4 Possible mechanisms for species migration
Because summer (xsSO4 2-, NO3 − and MSA) and sea-salt (Na+ and Mg2+) species are deposited in phase in the upper part of the core, we assume that the change in species relationship noted deeper in the core results from post-deposi-tioiial migration and not a change in deposition timing. Several different mechanisms could be responsible for the observed migration: (1) diffusion (both vapour phase and within solid ice), (2) gravitational movement, (3) interactions within and outside the ice lattice, (4) migration associated with strongly acidic (i.e. volcanic) layers, and (5) formation of insoluble salts (Wolff, 1996).
For MSA, migration may be in the up-core direction where it is first noted (1.5-2 m depth). This would rule out gravitational movement. Likewise, simple diffusion is unlikely, as peak shapes near the bottom of the detailed 24 m core section are still sharply defined, and new peaks have been formed where none previously existed. Wolff 1996 speculated that MSA may form an insoluble salt with one of the sea-salt cations, which would then allow continued diffusion, as the insoluble salt is a different species and would mainta in the gradient. This process would effectively deplete the original peak, and form a new one in the sea-salt peak area, similar to what is observed at Siple Dome. The feasibility of this process is hard to assess since there is little information on the low-temperature solubility of MSA salts (Wolff, 1996). in addition, the freezing point of Na+ or Mg2+ salts of MSA may be above the temperature of the ice, effectively removing MSA from the liquid phase. Again, there is little information on freezing-point curves for Na+ or Mg salts of MSA (Mulvaney and others, 1992). As the most pronounced MSA migration has been noted at sites with high sea-salt concentrations (Dolleman Island, FRIS, Siple Dome), it is imperative to understand the reactions between MSA and sea salts to determine if these are the main cause of migration. Another possibility is that MSA is located within veins at a eutectic composition, and therefore could move across an apparent gradient in bulk composition Mulvaney and others, 1992). Eutectic compositions of strong acids commonly freeze below 0°C, with many remaining liquid well below the temperature of Antarctic ice. This explanation, however, may not be effective in firn where ice grains have not compacted to form a completely interlinked network of veins, as in the upper 24 m of the Siple Dome core.
High summer biogenic SO4 2-. concentrations in Siple Dome summer layers appear to have a significant effect on NO3 − concentrations. The average summer SO4 2- maximum in the upper 24 m (6.10 μq 1−1) of the Siple Dome core is greater than all but the largest volcanic SO4 2- spikes (e.g. Tambora and AD 1259 events) recorded in Antarctic ice cores (Reference Dai, Mosley-Thompson and ThompsonDai and others, 1991; Dclmas and others, 1992; Lang-way and others, 1995) and Crccnland ice cores (Laj and others, 1993) over the last 1000 years. NO3 − migration from summer to winter layers is also seen (but not commented on) in Berkner Island (Wagenbach and others, 1994) and FRIS ice cores (Minikin and others, 1994), coastal sites with seasonal SO4 amplitudes comparable to Siple Dome's. At all three locations, NO3 − migration appears to be limited to either the preceding or following winter layer, with peaks forming where none previously existed. Therefore, as with MSA, migration of both acids appears to be limited by winter layers with high sea-salt concentrations. Mechanisms to explain this phenomenon have not been studied in detail, but may include decreased sticking probability of the HNO3 molecule due to increased acidity (Laj and others, 1993). (lonstant NO3 − migration (e.g. occurring every year below a certain depth) may be limited to coastal sites where there is alternation between more acidic summer and less acidic winter layers. Although wc can provide no definite cause for NO3 − migration, it is clear that NO3 − migration from volcanic layers cannot be ignored in the interpretation of changes in nitrogen atmospheric chemistry during volcanic periods.
The only other ice-core location where Mg2+ movement has been observed is Dolleman Island, where, as at Siplc Dome, sea-salt concentrations are high (Dolleman Island: ~12.6μeql −1 Na+, ~3.0μeql−1 Mg2+ (Mulvaney and others, 1992); Siple Dome: ~7.1 μeql −1 Na+, ~2.1 μeql −1 Mg2+). The formation of two distinct peaks on either side of an Na+ peak rules oui simple diffusion, which would tend to smooth the record, and gravitational movement of a grain-boundary liquid. Wolff (1996) suggested that there may be a limited number of cation sites on grain boundaries, and that as the grain grows and surface area decreases, the number of sites may decrease. As sea-salt particles (initially present as discrete particles) slowly dissolve, Na may preferentially occupy external sites, while Mg2+ is driven away from the high Na+ and CT region (Wolff, 1996). This mechanism is speculative, and more work on this problem is clearly-needed.
3.5 Implications for core interpretation
Migration of all three species discussed here appears to be limited to either the preceding or following seasonal layer. in the case of MSA and NO3 − , migration is essentially stopped by the high concentration of sea sail in the winter layer. A similar pattern is observed for MSA at Dolleman Island, where migration continued at least until pore close-off, but never went farther than one year (i.e. into the trough of the previous or following summer). in fact, deeper in the Dolleman Island core, MSA peaks became sharper, and summers became more and more depleted (Wölfl, 1996). The maximum extent of Mg migration appears to be one season, as with MSA and NO3 −. in most cases, Mg2+ appears to migrate only to the shoulders of Na peaks. Therefore, we would expect that interpretations based on data of greater than annual resolution will not be affected by Mg2+ migration.
To fully understand the observed chemical migration phenomena, two approaches are likely needed. First, continned collection of samples from a wide variety of locations in Antarctica, such as those planned as part of the International Trans-Antarctic Scientific Expedition (Mayewski and Goodwin, 1996), will provide a more detailed spatial picture of the conditions under which these phenomena occur. Second, laboratory experiments are needed to investigate the small-scale interactions of chemical species and ice. For example, information on the exact location of impurities with in the ice lattice and vapor-pressure effects would be useful in investigating species migration. While our results suggest that species migration is limited to less than one annual layer, an ultimate goal of ice-core paleoclimatic ret (instructions is to make interpretations on sub-annual scales. To enable us to do so with long time-series ice-core glaciochemical records in areas where species migration occurs, our understanding of these problems must improve.
Acknowledgements
Wc ank Q. Yang for field assistance, J.W.C. White for isotopir data, R. Alley for stratigraphie measurements, the Polar Ice Coring Office (D. Giles and D. Kahler) for recovering the core, Navy SqiiadronVXE-6 and Antarctic Support Associates. This research was supported by the Office of Polar Programs, U.S. National Science Foundation.