INTRODUCTION
Vast aeolian systems occur adjacent to west-to-east flowing rivers across the Southern Great Plains (SGP) (Fig. 1), have repeatedly reactivated in the Holocene, and are potentially significant sources of dust for adjacent metropolitan centers (e.g., Routson et al., Reference Routson, Overpeck, Woodhouse and Kenney2016; Bolles et al., Reference Bolles, Sweeney and Forman2019; Sweeney et al., Reference Sweeney, Forman and McDonald2022). This area where stabilized and active dune systems abound is characterized by moderate to high wind drift potential, with assumed threshold wind speeds of 5 to 6 m/s for aeolian entrainment (Schmeisser et al., Reference Schmeisser, Loope and Mason2010), though higher wind thresholds of ≥10 m/s may be needed on crusted or vegetated surfaces to initiate saltation (Bolles et al., Reference Bolles, Sweeney and Forman2019; Sweeney et al., Reference Sweeney, Forman and McDonald2022). Foundational studies indicate abundant aeolian sediment sources from preexisting aeolian deposits and active fluvial systems on the SGP (e.g., Holliday, Reference Holliday1995; Muhs, Reference Muhs2017). Often, sediment sources and availability are enhanced by a paucity of stabilizing vegetation (i.e., reduced below a coverage threshold of ~40%) that may occur with multiyear to decadal-scale severe droughts or megadroughts (e.g., Forman et al., Reference Forman, Oglesby and Webb2001; Mangan et al., Reference Mangan, Overpeck, Webb, Wessman and Goetz2004; Stahle et al., Reference Stahle, Fye, Cook and Griffin2007; Cook et al., Reference Cook, Cook, Smerdon, Seager, Williams, Coats, Stahle and Diaz2016). However, critical questions remain concerning whether dune reactivation on the Great Plains reflects a response to drought variability or more stochastic processes associated with grassland response to varying moisture availability, long stabilization times, grazing disturbance, and edaphic processes (e.g., Luna et al., Reference Luna, Parteli, Durán and Herrmann2011; Werner et al., Reference Werner, Mason and Hanson2011; Barchyn and Hugenholtz, Reference Barchyn and Hugenholtz2012; Hugenholtz and Koenig, Reference Hugenholtz and Koenig2014; Halfen et al., Reference Halfen, Lancaster and Wolfe2016).
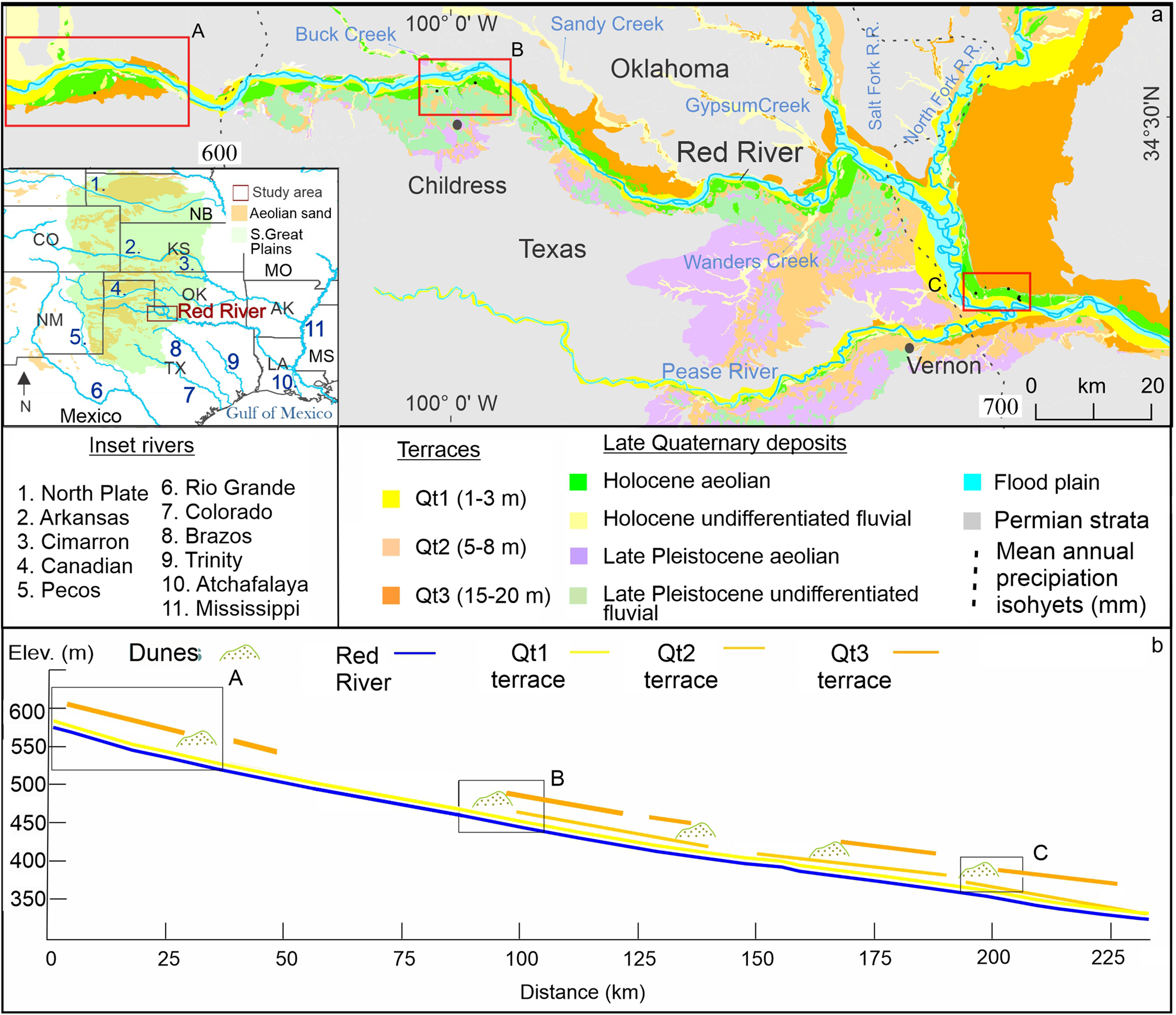
Figure 1. (a) Western Red River at Texas and Oklahoma border in the eastern edge of Southern Great Plains (inset) showing current channel; floodplain; Qt1, Qt2, and Qt3 terraces; and surficial Late Quaternary deposits. Dashed line indicates mean annual precipitation isohyets, with study area mostly between 600 and 700 mm. Red boxes labeled A, B, and C indicate study areas shown in Fig. 2. (b) Diagrammatic cross-section for the length of part a showing the Red River; Qt1, Qt2, and Qt3 terraces; and major dunes.
A significant source for aeolian sediments during periods of dune expansion or accretion may be from fluvial systems in this semiarid environment (e.g., Kocurek and Lancaster, Reference Kocurek and Lancaster1999; Lancaster and Tchakerian, Reference Lancaster, Tchakerian, Enzell, Wells and Lancaster2003; Wright et al., Reference Wright, Forman, Waters and Ravesloot2011; Halfen et al., Reference Halfen, Lancaster and Wolfe2016; Sankey et al., Reference Sankey, Caster, Kasprak and East2018). Specifically, braided reaches or broad exposed floodplains may originate source-border dunes on the adjacent floodplain or fluvial terraces (Draut, Reference Draut2012; Liu and Coulthard, Reference Liu and Coulthard2015; Sankey et al., Reference Sankey, Caster, Kasprak and East2018). Rivers can be also barriers to sand dune migration, as shown by the Colorado River impeding sand transport from the Mojave Desert (Muhs et al., Reference Muhs, Reynolds, Been and Skipp2003; Draut, Reference Draut2012). Flood deposits of dryland rivers can be aeolian sediment sources (Bullard and McTainsh, Reference Bullard and McTainsh2003; Sankey et al., Reference Sankey, Caster, Kasprak and East2018). The influence of abundant flood-derived sediments on aeolian source zones can persist tens to hundreds of kilometers downwind along a river (e.g., Muhs et al., Reference Muhs, Reynolds, Been and Skipp2003; Alizai et al., Reference Alizai, Carter, Clift, Van Laningham, Williams and Kumar2011). In turn, fluvial degradation and channel migration may perch dune fields above the active river channel. These aeolian systems may be partially or wholly isolated from riverine sediment sources, and with a fall in the water table, vegetation may shift to drier C4 grassland species and lower the threshold for dune reactivation with drying (e.g., Forman et al., Reference Forman, Oglesby and Webb2001; Mangan et al., Reference Mangan, Overpeck, Webb, Wessman and Goetz2004; Houser et al., Reference Houser, Bishop and Barrineau2015).
There is compelling evidence for multiple aeolian depositional events in the past 2000 yr for many dune fields adjacent to major drainages on the SGP (e.g., Forman et al., Reference Forman, Oglesby and Webb2001, Reference Forman, Marin, Gomez and Pierson2008; Werner et al., Reference Werner, Mason and Hanson2011; Halfen and Johnson, Reference Halfen and Johnson2013). However, there is considerable uncertainty surrounding the asynchrony of aeolian systems’ activity and times of stability across the SGP, reflecting a number of factors, including varying sediment supply and availability, threshold wind speeds, hydrologic and climatic variability, lagged aeolian system response, stratigraphic incompleteness, and the limited number of detailed chronostratigraphic studies spanning the Holocene (e.g., Holliday, Reference Holliday2001; Forman et al., Reference Forman, Marin, Gomez and Pierson2008, Reference Forman, Tew-Todd, Mayhack, Wiest and Money2022; Halfen, Reference Halfen2012; Halfen and Johnson, Reference Halfen and Johnson2013; Halfen et al., Reference Halfen, Lancaster and Wolfe2016). Dune fields adjacent to the Arkansas River in west Kansas revealed a sequence of aeolian sands intercalated with weak buried soils that yielded optically stimulated luminescence (OSL) ages reflecting sand sheet accretion at ca. 1490 to 1250, 430, 380 to 320, 180, and 70 yr ago (Forman et al., Reference Forman, Marin, Gomez and Pierson2008). Three broad periods of aeolian accretion at ca. 2100 to 1800, 1000 to 900, and 600 to 70 yr were inferred for the Hutchinson dunes in east-central Kansas based on OSL dating of strata (Halfen et al., Reference Halfen, Johnson, Hanson, Woodburn, Young and Ludvigson2012). In contrast, large, stabilized dune ridges on the second terrace of the Cimarron River yielded OSL ages between 880 and 770 yr (Lepper and Scott, Reference Lepper and Scott2005). Significant aeolian erosion on upland surfaces adjacent to the Cimarron River bend area (in Kansas and Oklahoma) is indicated by large deflation hollows infilled by sand sheet–type strata spanning from 810 to 460 yr (Werner et al., Reference Werner, Mason and Hanson2011). OSL dating of paleosurfaces preserved beneath fence-line deposits near the Canadian River in northwest Oklahoma revealed aeolian deposition at ca. 215 yr ago, before cultivation, with overlying sands from the twentieth century Cordova et al. Reference Cordova, Porter, Lepper, Kalchgruber and Scott2005). Soils and 14C ages from the Southern High Plains indicate spatial discontinuous occurrence of sand sheets ca. 1000 to 500 cal yr BP, with stability soon after and additional sand sheet deposition at 500 to 300 cal yr BP (Holliday, Reference Holliday2001). Radiocarbon ages of buried A horizons at the Muleshoe dune field in west Texas provide maximum limiting ages on soil burial by aeolian sand at ca. ~1300, 750 to 670, and ~500 cal yr BP (Holliday, Reference Holliday2001). The Mescalero sand sheet in southeastern New Mexico buried a subjacent paleosol and yielded a basal OSL age of 620 yr (Hall and Goble, Reference Hall and Goble2012). At the surface, coppice and parabolic dunes abound and may reflect anthropogenic disturbance since the nineteenth century (Hall and Goble, Reference Hall and Goble2012). This study focuses on deciphering the fluvial and aeolian geomorphic and stratigraphic record along the upper reach of the Red River, west of the confluence with the Pease River (Figs. 1 and 2). We delineate the possible fluvial–aeolian depositional pathways for formation of source-border dune fields with climate variability in the past ca. 600 yr. Geoprobe cores, 5 to 15 m long, allow close-interval OSL dating of quartz grains through surface-forming aeolian deposits and underlying terraces that reveals significant time (103 to 104 yr scales) unconformities.

Figure 2. Geomorphic forms for study areas A–C as shown on Fig. 1. Subfigures A1, B1 and C1 are Google Earth images derived from aerial photographs (May 2016). Subfigures A2, B2, and C2 are line drawings showing distribution of aeolian landforms for the most recent blowout dunes (yellow) and older parabolic dunes (orange) accreted from winds from the west to northwest, with most common sinuous ridges (dark brown) showing the north–south sense of these oldest landforms. The directionality of aeolian landforms is detailed by the inset directional roses, with color (yellow, orange, and dark brown) reflecting corresponding mapped aeolian landforms (blowout dune, parabolic dune, and sinuous ridge). Subfigures A3, B3, and C3 show detailed mapping of terraces and surficial deposits in reference to location of stratigraphic sites and Geoprobe cores (RR18- or RR16-) from Fig. 1.
CLIMATIC AND GEOMORPHIC CONTEXT OF THE WESTERN RED RIVER, TX-OK BORDER
The Red River flows across a significant moisture gradient, with mean annual precipitation (MAP) of 400 mm near its headwaters at 1050 m above sea level (m asl) elevation in west Texas and adjacent New Mexico to 1500 mm MAP at its point of discharge in eastern Louisiana into the Atchafalaya River at 25 m asl (Bertrand and McPherson, Reference Bertrand and McPherson2018). In west Texas, this river flows across Permian carbonate lithologies, gray sandstones, and red shales and sandstones; suspended sediment from these last lithologies is the presumed source of the red color. The high variance in discharge of the Red River exemplifies twentieth- and twenty-first-century climate variability on the SGP, with often-dry bed conditions during droughts between 2008 and 2014; extreme flood events in wetter years, as in 2015; and longer dry periods projected by 2050 (Qiao et al., Reference Qiao, Zou, Gaitán, Hong and McPherson2017; Bertrand and McPherson, Reference Bertrand and McPherson2018). Discharge of the western Red River becomes intermittent during drought conditions, with net transmission loss through channel infiltration (Bertrand and McPherson, Reference Bertrand and McPherson2018; Huggins et al., Reference Huggins, Gleeson, Kummu, Zipper, Wada, Troy and Famiglietti2022; Zipper et al., Reference Zipper, Popescu, Compare, Zhang and Seybold2022) and broad exposure of the valley train for aeolian reworking.
The wind climate of the western Red River, defined in this study using wind velocity and direction data for the Childress Municipal Airport data between 1943 and 2019 (Fig. 3A), shows significant seasonal variability (Fig. 3B). Winter and early spring wind directions are from northwest to west, with the strongest wind drift potential (Fryberger, Reference Fryberger and Mckee1979; Yizhaq et al., Reference Yizhaq, Xu and Ashkenazy2020) in March and April (Fig. 3A and B). In contrast, late spring through summer wind directions are mostly from the south, with lower wind drift potential values (Fig. 3B) Thus, the lowest discharge for the Red River (Fig. 3C), with maximum exposure of channel, bar, and floodplain surfaces, appears coincident with the highest monthly average wind drift potential for January to April (Fig. 3B), which are conditions conducive for aeolian transport from fluvial surfaces and accretion of source-border dune fields on adjacent terraces.

Figure 3. Wind climate for Childress Municipal Airport site with data from February 1, 1943, to August 27, 2019 (https://www.ncdc.noaa.gov/cdo-web/datasets/GHCND/stations/GHCND:USW00023007/detail). (A) Wind rose. (B) Wind drift potential (DP), resultant drift potential (RDP), and resultant drift direction (RDD); the lower part of B shows monthly distribution of drift potential values in vector units (VU) (Fryberger, Reference Fryberger and Mckee1979; Yizhaq et al., Reference Yizhaq, Xu and Ashkenazy2020). (C) Average monthly discharge for U.S. Geological Survey Stream Gauge 07299540 for the Prairie Dog Town Fork, Red River near Childress, TX, for the period April 1965 to April 2020 (https://waterdata.usgs.gov/nwis/inventory/?site_no=07299540&agency_cd=USGS).
The western Red River is a meandering river with a broad floodplain and numerous Late Quaternary flights of geomorphologically distinct river terraces (Frye and Leonard, Reference Frye and Leonard1963; Edwards, Reference Edwards2016). These terraces were mapped by Frye and Leonard (Reference Frye and Leonard1963) for the length of the Red River and major tributaries, and are named, from highest to lowest: Hardeman (~32 m), Ambrose (~10 m), and Cooke (~5 m). There are no direct ages for these terraces; inferred late Pleistocene ages are based on molluscan biostratigraphy and broad correlation with midwestern U.S. glacial stages such as Kansan and early and late Wisconsinan, respectively (Frye and Leonard, Reference Frye and Leonard1963).
In contrast, the terraces and meander belts of the lower Red River and Mississippi River valleys have been the focus of numerous studies, particularly near the confluence with the Atchafalaya River (Autin Reference Autin1996; Tornqvist et al., Reference Tornqvist, Kidder, Autin, van der Borg, de Jong, Klerks, Snijders, Storms, van Dam and Wiemann1996; Aslan and Autin, Reference Aslan and Autin1998; Shen et al., Reference Shen, Törnqvist, Autin, Mateo, Straub and Mauz2012). Five major fluvial allostratigraphic units—the Upland, Intermediate, Prairie, and Deweyville complexes and Holocene meander belts—have been recognized in the lower Red River and Mississippi valleys (LMV); and adjacent Gulf Coast Plain (Blum and Valastro, Reference Blum and Valastro1994; Autin, Reference Autin1996; Blum and Aslan, Reference Blum and Aslan2006; Shen et al., Reference Shen, Törnqvist, Autin, Mateo, Straub and Mauz2012). The Upland Complex is the oldest and highest allostratigraphic unit, with an inferred age of late Tertiary to Early Pleistocene and may preclude influences from Pleistocene glaciations (Autin, Reference Autin1996). A younger allostratigraphic member is the Early to middle Pleistocene Intermediate Complex expressed in the lower Red River Valley as the Montgomery terrace, which is highly dissected (Fisk, Reference Fisk1938; Autin, Reference Autin1996). The Prairie and Deweyville Complexes span from the late Pleistocene to the Holocene and are characterized by widespread fluvial aggradation of meandering streams (Autin, Reference Autin1996). The Prairie Complex along the lower Red River deltaic plain yielded OSL ages of 91 ± 9 and 104 ± 10 ka and 111 ± 10 ka for the higher terrace at the Avoyelles Prairie site (Shen et al., Reference Shen, Törnqvist, Autin, Mateo, Straub and Mauz2012). In most localities in the LMV, the Prairie Complex yielded OSL ages between ca. 135 and 70 ka, a time of shoreline progradation with high and stable sea level during Marine Oxygen Isotope Stage 5 (MIS 5) (Shen et al., Reference Shen, Törnqvist, Autin, Mateo, Straub and Mauz2012). Two additional OSL ages of ca. 220 and 215 ka from the Prairie Complex indicate a possible earlier initiation age, during MIS 7, although this association has been questioned (Otvos, Reference Otvos2013). The Deweyville Complex/Formation reflects net fluvial aggradation in the middle to late Wisconsinan (Autin, Reference Autin1996). A pivotal study with detailed mapping and associated OSL ages for high, well-preserved braid belts for the middle and lower LMV show fluvial aggradation ca. 64 to 11 ka in equilibrium with a steeper river gradient during the last glacial maximum (LGM) (Rittenour et al., Reference Rittenour, Blum and Goble2007). This fluvial depositional unit is widespread along rivers and tributaries in central and southern Texas (Blum et al., Reference Blum, Toomey and Valastro1994; Blum and Aslan, Reference Blum and Aslan2006), contains late Pleistocene fauna with 14C ages of 26 to 20 ka old (Lundelius et al., Reference Lundelius, Bryant, Mandel, Thies and Thoms2013, Reference Lundelius, Thies, Graham, Bell, Smith and DeSantis2019) and OSL ages between 44 and 8.6 ka (Meier et al., Reference Meier, Nordt, Forman and Driese2013). Studies of the Prairie and Deweyville Complexes and correlative deposits indicate that the fluvial incision in response to sea-level fall extends at least 500–600 km upstream from the current shoreline (Blum and Aslan, Reference Blum and Aslan2006; Rittenour et al., Reference Rittenour, Blum and Goble2007; Shen et al. Reference Shen, Törnqvist, Autin, Mateo, Straub and Mauz2012). Holocene meander belts abound in the floodplain of the LMV, with a majority deposited post 5.4 ka, a period of relatively stable global sea level (Aslan and Autin, Reference Aslan and Autin1998). Late Holocene flood records for the middle Mississippi valley, near St. Louis, indicate periods of sustained flooding during the seventeenth and early eighteenth centuries, with anthropogenic impacts starting in nineteenth century (Munoz et al., Reference Munoz, Gruley, Massie, Fike, Schroeder and Williams2015, Reference Munoz, Giosan, Therrell, Remo, Shen, Sullivan, Wiman, O'Donnell and Donnelly2018).
METHODS
Landform analysis for the upper Red River Valley
A landform analysis for the upper Red River basin was completed that shows flights of fluvial terraces often covered by aeolian sediments (Figs. 1, 2, and 4). Geomorphic mapping identified a variety of aeolian and fluvial landforms, including the active floodplain, three terraces sets at distinct heights, blowout and parabolic dunes, and sinuous ridges (Figs. 2 and 4). Surficial characteristics of landforms were revealed through analysis of multiple years of aerial photographs from the 1980s to 2020s from Google Earth Pro. Mapping was also aided by partial aerial photograph coverage from the 1930s, a time of less surface disturbance (Bolles et al., Reference Bolles, Forman and Sweeney2017), topographic maps, digital elevational models (1 and 10 m resolution), soil series maps from the Natural Resource Conservation Service, and field-based land surveys and stratigraphic studies. Mapped fluvial terraces were traced by elevation using digital elevation models (https://topobuilder.nationalmap.gov; https://www.freemaptools.com/elevation-finder.htm) and field assessments. Many terraces were physically traced in the field (Fig. 4) and intimately associated with stratigraphic localities to better understand fluvial landform height and the nature of aeolian cover. This mapping identified three Quaternary terraces from lowest to highest—Qt1, Qt2, and Qt3—with the respective height ranges of 1 to 3, 5 to 8, and 15 to 20 m; these were assigned the respective informal names Luna, Vernon, and Childress (Figs. 1 and 2). Although, many terrace heights may be exaggerated by the later addition of meters of aeolian sediments, the Geoprobe cores used in this study provided needed data on aeolian cover thickness and age to better assess terrace elevation with respect to the current Red River level (RRL).

Figure 4. (A) Broad Qt1 terrace at about 2 m above Red River inset into degraded slope of Qt 2 terrace. (B) Qt1 terrace at 2 m above and incised by the Red River. (C) Blowout dune on Qt1 terrace. (D) Hummocky surface of stabilized dunes over Qt1 and Qt2 terraces.
Sedimentary and stratigraphic analysis
The stratigraphic record of fluvial and aeolian processes and periods of landscape stability, as indicated by buried soils, was delineated through the study of four 1- to 3-m-high stratigraphic sections and six 5- to 14-m-long Geoprobe cores. Sedimentary sequences are characterized by numerous distinct depositional units of aeolian and fluvial sand, often with paleosols demarking the unit top. Usually, aeolian and fluvial stratigraphic successions represent a conservative record of depositional periods and associated hiatuses because of the unknown completeness associated with erosion and pedogenesis in terrestrial settings (e.g., Forman et al., Reference Forman, Tew-Todd, Mayhack, Wiest and Money2022). Thus, multiple sections and sequences of cores were taken across terraces and were analyzed to capture some of the spatial and temporal variability of fluvial and aeolian processes in the late Pleistocene and Holocene for the upper Red River. Sections and Geoprobe cores were studied with attention to sedimentologic and pedogenic details, including soil structure and texture, carbonate morphologies, Munsell color changes, variability in bed thickness, nature of bed contacts, carbonate content, and the associated granulometry (Fig. 5). Attention was focused on bedding planes and unit contacts to assess whether there were hiatuses in deposition, sometimes indicated by the presence of a buried soil or localized bioturbation. The recognition of a buried soil is pivotal, because this stratigraphic marker probably reflects landscape stability associated with relatively mesic conditions. We used well-vetted soil stratigraphic and geomorphic approaches (Birkeland, Reference Birkeland1999; Schoeneberger et al., Reference Schoeneberger, Wysocki, Benham and Staff2012). All soil Munsell colors are assessed in the moist state. Buried soils show clear signs of rubification and secondary accumulation of clay and silt, and in places there is evidence for precipitation of pedogenic carbonate. Aeolian stratigraphic units representing discrete depositional events were defined by either bounding buried soils or sedimentologic characteristics (Fig. 5). Granulometry was determined using a Malvern Mastersizer 2000 laser diffractometer with particle size limits set by Folk (Reference Folk1980) to assist in determining pedosedimentary facies (Table 1).

Figure 5. Ternary diagrams showing the distribution of silt and coarse and medium sand for fluvial and aeolian facies for Red River Holocene and late Pleistocene sediments.
Table 1. Pedo-sedimentary facies for Red River cores showing aeolian and fluvial deposits and pedogenesis.

OSL dating of aeolian and fluvial sediments
Strata were sampled for OSL dating after assessment of the pedosedimentary context (Figs. 6–8). At least one OSL sample was retrieved from each stratigraphic unit, dependent on unit thickness. Sampling in the field and laboratory avoided levels with diagenetic and pedogenetic alterations and favoring primary depositional sediments. These OSL samples were taken from stratigraphic sites using light-secure 4-cm-diameter and 13-cm-long sections of black ABS pipe hammered into the desired sampling level. OSL sampling for Geoprobe cores was completed under safelight conditions (Na-vapor bulb) within the Baylor Geoluminescence Dating Research Laboratory.

Figure 6. Stratigraphic sections (A) Pivot A and B Sites. (B) Mike Site adjacent to River Red. (C) Hollis Site showing recent aeolian deposition over fluvial sediments near river level. FS, fine sand; MS, medium sand; OSL, optically stimulated luminescence; SI, silt.

Figure 7. Stratigraphy of Geoprobe cores RRCOR16-1 and RRCOR16-3 with associated optically stimulated luminescence (OSL) ages, granulometry, and pedogenic morphologies. Particle size abbreviations: CLY, clay; FS, fine sand; MS, medium sand; SI, silt; VFS, very fine sand.

Figure 8. Stratigraphy of Geoprobe cores RR18-OK1, OK2, OK3, and OK7 with associated optically stimulated luminescence (OSL) ages, granulometry, and pedogenic morphologies. Particle size abbreviations: CLY, clay; FS, fine sand; MS, medium sand; SI, silt; VFS, very fine sand.<TYPESETTER: Please change part labels (a–d) to uppercase (A–D) in this figure.>
Single-aliquot regeneration (SAR) protocols (Murray and Wintle, Reference Murray and Wintle2003; Wintle and Murray, Reference Wintle and Murray2006) were used to estimate the equivalent dose (D e) of quartz fractions of a certain size range for 33 to 76 separate aliquots (Table 2). Each aliquot contained ~20 to 80 quartz grains corresponding to ~1 mm circular diameter of grains adhered (with silicon) to a 0.98-cm-diameter circular aluminum disk. The separation of quartz fractions for a specific grain-size range (e.g., 63–100 μm) followed the procedure outlined in Marin et al. (Reference Marin, Forman, Todd, Mayhack, Gonzalez and Peng2021). Note that all OSL ages are calculated with respect to recent corrections in beta source collaborations (Autzen et al., Reference Autzen, Andersen, Bailey and Murray2022). Details of OSL dating are provided in Appendix 1 in the Supplementary Material.
Table 2. Optically stimulated luminescence (OSL) ages on quartz grain extracts and associated data for aeolian and fluvial sediments from Red River study area, Texas and Oklahoma.

a Aliquot number used for single-aliquot regeneration (SAR). Equivalent dose calculated using the central age model of Galbraith and Roberts (Reference Galbraith and Roberts2012).
b D e (equivalent dose) calculated on a pure quartz fraction with about 40–100 grains/aliquot and analyzed under blue-light excitation (470 ± 20 nm)
by SAR protocols (Murray and Wintle, Reference Murray and Wintle2003; Wintle and Murray, Reference Wintle and Murray2006). The central age model of Galbraith et al. (Reference Galbraith, Roberts, Laslett, Yoshida and Olley1999) was used to calculate D e when overdispersion (OD) values are <20%. The minimum age model was used with OD values >20% to resolve the youngest D e population.
c OD values reflect precision beyond instrumental errors; OD values of ≤20 indicate low dispersion in D e values with a lognormal distribution (Galbraith and Roberts, Reference Galbraith and Roberts2012).
d U, Th, Rb, and K2O content analyzed by inductively coupled plasma–mass spectrometry (ICP-MS) analyzed by ALS Minerals Incorporation, Reno, NV, USA.
e From Prescott and Hutton (Reference Prescott and Hutton1994) and Liang and Forman (Reference Liang and Forman2019).
f Systematic and random errors calculated in a quadrature at 1 SD. Datum year is AD 2010. Age computation through the Luminescence Dose and Age Calculator (LDAC) at: https://www.baylor.edu/geosciences/index.php?id=962356 (Liang and Forman, Reference Liang and Forman2019).
RESULTS
Fluvial and aeolian depositional record of the upper Red River Valley
Three broad levels of fluvial terraces were identified immediately to about 15 to 20 m above and adjacent to the western Red River (Figs. 1, 2, and 4). These terraces occur from highest to lowest above the seasonally lowest water level (during fall and winter) as Qt3 (15–20 m), Qt2 (5–8 m), and Qt1 (1–3 m). The lowest terrace discerned was about 0.5 m above RRL, inset into the Qt1 terrace in places. Modern flood debris and abandoned channels, point bars, natural levee ridges and bars, and swale topography were common on this lowest terrace and evident on the Qt1 terrace in places, such as the Mike Site (Fig. 2A).
Aeolian deposits are common on terraces and extend the apparent height of terraces by up to 10 m in places and protect older terraces from subsequent erosion (Figs. 7 and 8). These aeolian-covered terraces were targets for extracting Geoprobe cores to discern the age and stratigraphic relation between fluvial and aeolian processes. Also, stratigraphic sections were studied along the banks of the Red River and barrow pits (Figs. 6–8). The dune fields adjacent to the Red River are mostly stabilized and often well vegetated and occur preferentially on the inside of meander bends, where the supply of sediments may be enhanced for aeolian transport from extensive bars and wider channels (Figs. 1 and 2). Aeolian landforms are well expressed at the three study sites (Sites A, B, and C; Fig. 2), and reveal a complex diachronous relation between aeolian deposits and the underlying terraces (Figs. 7 and 8). Sites A and B show superposition relations among recent blowout dunes, older parabolic dunes, and the oldest aeolian landforms, sinuous ridges on the Qt2 and Qt3 terraces (Fig. 2). The youngest apparent landforms are blowout dunes and are well expressed riverward in stabilized dune fields at Sites A and B (Fig. 2). Blowout dunes show fresh, less-vegetated surfaces, with elongate erosion depressions of 5 to 40 m in length orientated northwest and southeast, indicating formation by winds from the west to northwest, which are dominant wind directions in the winter and early spring (Fig. 3). These blowout dunes crosscut larger and clustered parabolic dunes, which vary in length from ~50 to 200 m, often with asymmetric arms. The orientation of these parabolic dunes indicates dominant formation by winds from the west to northwest, consistent with the highest wind drift potential during March and April (Fig. 3). The apparent, oldest aeolian landform, confined to the Qt2 and Qt3 terraces, are parallel to subparallel sinuous ridges, some >1 km long. These ridges have broad crests, 10 to 30 m wide, with slope angles of 5° to 10° and no discernible consistent slope of asymmetry. The long-axis orientation of these degraded aeolian landforms is north to south, with a wind sense of formation from the west to east plane.
Pedosedimentary facies for Red River fluvial and aeolian sediments
Four pedosedimentary facies (1, 2, 3, and 4) were defined to better characterize the sedimentologic and pedogenic variability exposed in stratigraphic sections and the Geoprobe cores (Table 1). These facies are the basis for defining depositional sequences, lacunae and to infer paleoenvironments (e.g., Miall Reference Miall1996; Forman et al., Reference Forman, Tew-Todd, Mayhack, Wiest and Money2022). Facies are defined by the associated granulometry (Fig. 5), bedforms, contact between units, and pedogenic horizons (e.g., Bs, Bw, Bt, and Bk). Facies 1 is an orange to yellow, moderately to poorly sorted, coarse to fine sand with common small pebbles (0.5–2.0 cm in diameter), granules, and granule-size clay- and silt-rich intraclasts. This facies appears massive in cores and in places shows clear centimeter-scale bedding, with common orange to yellow iron staining. The upper boundary of this facies is often demarked by a truncated argillic or cambic horizon, reflecting subaerial exposure, landform stabilization, and subsequent erosion. These sediments are dominated by coarse sand and granules (Fig. 5), reflecting a high- to moderate-energy fluvial depositional environment associated with channel and channel margin processes. Facies 2 is finer grained than Facies 1, being a moderately well-sorted, silty to slightly silty sand (Fig. 5). It (Table 1) has a distinctive orange to yellowish-brown color with intercalated weakly to moderately developed buried soils, some with carbonate (stages 1–2), argillic and cambic morphologies. Facies 2 is associated with low-energy fluvial environments such as floodplains or levees.
Common aeolian sediments adjacent to the Red River are represented by Facies 3 and 4. Facies 3 is a mostly massive, light yellow to gray, moderately sorted, silty fine sand to sandy silt (Fig. 5, Table 1). It is often pedogenically modified and exhibits carbonate filaments (stage 1) and weak cambic and argillic morphologies, with a well-developed buried soil demarking the upper boundary. Facies 3 can exhibit millimeter-scale subhorizontal to horizontal bedding and bedding remnants disrupted by common burrowing. It is interpreted as a sand sheet environment with intermittent deposition and pedogenesis. Facies 4, another aeolian sediment, is often coarser than Facies 3 and is composed of yellow to gray, very well sorted, medium to fine sand with less occurrence of silty sand (Fig. 5). In places it is massive, very well sorted, medium to fine sand, but can show centimeter-scale bedding in places. Facies 4 reflects interdunal and dune depositional environments, possibly associated with parabolic and barchanoid forms.
Pivot A and Pivot B Sites (34.55022°N, 100.54395°W, 573 m elevation, 21 m above the Red River)
The Pivot A and B Sites shows aeolian sediments over Qt2 or Qt3 terraces (Figs. 1 and 2A). Two sections studied in a local barrow pit, Pivot A and Pivot B, are about 10 m apart and expose the upper aeolian sand (Fig. 6). The Pivot A Site exposes the oldest stratigraphy identified, with a basal OSL age of 3295 ± 90 yr (BG4374) (Fig. 6). This basal aeolian unit (1) is massive, at least 1.2 m thick, and is composed of well-sorted, very fine to silty fine sand (Facies 3) with a cambic horizon developed in the upper 0.5 m. A similar, slightly coarser fine sand (Unit 2) unconformably overlies this lowest unit (3), with a truncate Ab at the top of Unit 2. The uppermost unit (3) is a very well sorted, fine sand with millimeter- to centimeter-scale horizontal to subhorizontal bedding (Facies 3). Units 2 and 3 yielded the respective OSL ages of 550 ± 20 yr (BG4540) and 470 ± 20 yr (BG4373). Pivot B, a correlative section 10 m to the north of the Pivot A Site (Fig. 6), exposed the upper two units (2 and 3) with corresponding OSL ages of 475 ± 20 yr (BG4374) and 510 ± 20 yr (BG4375). These sections reflect a phase of sand sheet deposition commencing at ca. 550 to 500 yr separated by an ~50 yr period of relative landscape stability with pedogenesis forming a cambic horizon, with renewed aeolian deposition ca. 450 to 475 yr ago (Fig. 6).
Mike Site (34.5808°N, 100.5852°W, 559 m elevation, 1 m above the Red River)
The Mike Site exposes the stratigraphy at the lowest cutbank adjacent to the Red River (Fig. 6B). This site shows recent fluvial sediments, part of the floodplain, and the lowest identified tread, which forms the base of the riser for terrace Qt1 and rises 1 to 2 m above the Red River. The basal unit at this site is Facies 2–type sediment, with millimeter- to centimeter-scale horizontal beds of silty clay reflecting floodplain deposition, and yielded an OSL age of 170 ± 15 yr (BG4370). The overlying units (2 and 3) are composed of Facies 2–type sediment, fining upward from a medium to coarse sand at the base of Unit 4 to a medium to fine sand, perhaps reworked aeolian sand. OSL ages on quartz grains from the top of this section of 30 ± 5 (BG4371) indicate deposition in the late twentieth century (Fig. 6B).
Hollis Site (34.5775°N, 99.95365°W, 471 m elevation, 1.5 m above the Red River)
The Hollis Site is a section exposed along the banks of the Red River with its base at the current floodplain (Fig. 6C). This is a fining upward sequence from an overbank sediment (Facies 2) at the base capped by ~3 m of aeolian sand (Facies 3) with a clear cambic paleosol at the top of Unit 2, buried by 40 cm of recent aeolian sand (Facies 2). OSL ages on quartz grains of 220 ± 15 (BG4376) and 195 ± 20 (BG4377) from Unit 2 indicate deposition in the nineteenth century. OSL ages on the overlying aeolian sand (Unit 3) of 15 ± 5 (BG4378) demonstrate recent deposition in the past decade from the adjacent Red River floodplain (Fig. 6C).
Core RRCOR16-1 (34.54944°N, 100.0081°W, 487 m elevation, 11.5 m above the Red River)
Core RRCOR16-1 was taken from terrace 2 surface covered by Pleistocene/Holocene aeolian deposits (Figs. 1 and 4). This core contains over 4 m of aeolian sediment, mostly of Facies 3, spanning from ca. 23.3 ka to 30 yr ago (Fig. 7, Table 2). These aeolian sands bury an argillic and carbonate-rich soil, developed in fluvial deposits (Facies 2), that yielded an OSL age of 80,900 ± 3980 yr (BG4848). This oldest aeolian sediment (Unit 2) is a moderately well-sorted, medium sand that fines upward to a sandy silt, transitioning to a truncated Bw horizon developed at the unit top. This cover sand was deposited between ca. 23 and 14 ka. Unconformably overlying Unit 2 is a finer, cover loam aeolian sediment (Unit 3) with >60% silt and clay that yielded OSL ages between ca. 10 and 8 ka (Fig. 7). Aeolian sedimentation in Unit 4 renewed ca. 6.9 ka with emplacement of a moderately sorted, silty sand until ca. 4 ka, transitioning to a cummulic buried A horizon that marks the top of Unit 4. The uppermost aeolian, Unit 5, is 0.5-m-thick, moderately well-sorted, fine to medium sand to silty-fine sand with affinities to Facies 4. Quartz grains from the base of Unit 5, 40 cm below the current surface, returned an OSL age of 70 ± 5 yr (BG4842), and grains 15 cm below the surface gave an OSL age of 30 ± 5 yr (BG5018) indicating recent reworking of sediments.
RRCOR16-3 (34.56222°N, 99.94806°W, 491 m elevation, 23 m above the Red River)
Core RRCOR16-3 was taken on the downwind edge of mapped Holocene aeolian deposits, which appears to be a stabilized, vegetated paleo–dune field that buried the Qt2 or Qt3 terrace (Figs. 2 and 7). The basal sediments (Units 1 and 2) of this core from 7.9 to 4.7 m deep revealed a distinct reddish-yellow, moderately to poorly sorted, medium to coarse, granular-rich subrounded sand with rare small, rounded pebbles, characteristic of fluvial Facies 1 (Fig. 7). Quartz grains from unit 2 near the base of this core yielded an OSL age of 19,835 ± 1020 yr (BG4814), and grains 15 cm below the top of Unit 2 returned an age of 17,725 ± 970 (BG4390). Unconformably overlying Unit 2 is a massive, finer sediment, sandy silt to silty sand (Unit 3), with a paleosol demarking the upper boundary. A stratigraphic sequence of six OSL ages from Unit 3 indicates conformable sand sheet deposition (Facies 3) between ca. 13.0 and 3.3 ka, with the upper boundary between Units 3 and 4 at a truncated buried A horizon. The two uppermost Units, 4 and 5, are sandier than subjacent sediments (Facies 4), separated by an A horizon that spans ca. 500 yr (Fig. 7).
Core RR18-OK1 (34.22833°N 99.09911°W, 356 m elevation, 13.0 m above the Red River)
Core RR18-OK1 was taken within a stabilized dune field at a prominent meander bend in the Red River with a broad floodplain (Fig. 4C). This core revealed >7 m thickness (Units 1–3) of a fining upward sequence of fluvial sediments (Facies 1 and 2) with bounding ages of ca. 84.6 and 72.2 ka (Fig. 8A). Overlying these fluvial sediments are four separate aeolian sand units (Units 4–7; Fig. 8A). The lowest aeolian unit (4) is a moderately sorted, massive, silty sand that yielded an OSL age of 60,210 ± 3010 yr (BG4960). The overlying Unit 5 is a moderately well-sorted, medium to fine sand, with the upper ~1 m showing two compound, buried Bw horizons. Quartz grains from Unit 6, defined by bounding paleosols (Fig. 8A), returned an OSL age of 14,290 ± 730 yr (BG4959). The uppermost 2.7 m that defines the surface dunes (Unit 7) was a moderately well-sorted, medium to fine sand that gave the bounding OSL ages of 290 ± 15 yr (BG4958) and 185 ± 10 yr (BG4957) (Fig. 8A).
Core RR18-OK2 (34.240706°N, 99.1159436°W, 361 m elevation, 18.0 m above the Red River)
Core RR18-OK2 was taken about 2 km southwest of RR18-OK1 at the northern margin of the mapped paleo-dune field (Fig. 2C). This core revealed over 9 m of a fining upward sequence of fluvial sediment (Facies 1 and 2) succeeded by over 4 m of aeolian sand with two intercalated buried soils (Fig. 8B). The lowermost unit (1) is a high-energy fluvial facies (1) with abundant clay-rich intraclasts, granules, and common small, rounded pebbles. Quartz grains from the base and the top of the lowest unit (1) returned OSL ages of 84,640 ± 4470 yr (BG4804) and 70,800 ± 3290 yr (BG4802), respectively, and appear correlative with Units 1–3 of core RR18-OK1 (Fig. 7). In contrast, core RR18-OK2 has overlying younger fluvial sediments, Units 2 and 3, with respective OSL ages of 30.8 ka and 29.6 to 12.8 ka (Fig. 8B). Fluvial sediments of Unit 3 are bounded by two prominent paleosols. The overlying moderately sorted, sandy silt (Unit 4) is a cover sand–like deposit laid down ca. 6.7 ka. The top of Unit 4 is designated by a truncated cambic horizon, buried by a moderately sorted, silty fine sand, similar to subjacent Unit 3, that returned an OSL age of 470 ± 25 yr (BG4795). The uppermost aeolian silty sand (Unit 6), nearly 3 m thick, yielded the corresponding bottom and top OSL ages of 400 ± 20 yr (BG4794) and 410 ± 20 yr (BG4793).
Core RR18-OK3 (34.22237°N, 99.09833°W, 352 m elevation, 9.0 m above the Red River)
Core RR18-OK3 was taken at the riverward edge of the stabilized dune field, closest to a possible fluvial sediment source for adjacent dunes (Fig. 2C). This core showed 10.5 m of aeolian sands over 1.5 m of fluvial sediment (Fig. 8C). The lowest fluvial sediment (Unit 1) is characteristic of Facies 1, with a coarsening upward sequence with common silty-clay intraclasts. Quartz grains from a fine sand lens returned an OSL age of 4005 ± 215 yr (BG4865). The overlying Unit 2 is a finer-grained overbank deposit (Facies 2) with compound argillic and carbonate (stage 1) buried soil horizons. OSL ages of 2790 ± 140 yr (BG4884) and 1760 ± 90 yr (BG4983) from the base and top of Unit 2 constrain timing of aggradation. Thus, fluvial aggregation commenced at least 4.0 ka and stabilized at ca. 1.6 ka, as indicated by buried soils (in Unit 2), at about 1 to 2 m above the present RRL (Fig. 8C).
Unconformably above the basal fluvial sediments are multiple, Facies 3–type sediments of a sand sheet, Units 3–6. These units are characterized by individual fining upward sequences from a slightly silty sand to sandy silt, reflecting stabilization with the occurrence of truncated buried soil at the upper boundary of units (Fig. 8C). Five OSL ages from the base of Units 3–5 indicate that these sand sheet sediments, separated by cambic buried soil horizons were deposited during three depositional phases at ca. 780 to 750 yr, 500 to 450 yr, and 425 to 400 yr (Fig. 8C). The uppermost, Unit 6, is 4 m of well-sorted, fine to medium aeolian sand (Facies 4), which formed the current stabilized dune landscape, and yielded bounding OSL ages of 425 ± 15 yr (BG4859) and 405 ± 15 yr (BG4858).
Core RR18-OK7 (34.22237°N, 99.16671°W, 357 m elevation, 7 m above RRL)
Core RR18-OK7 was taken from a proximal dune field at a prominent bend in the Red River (Fig. 2C). This core revealed 10.6 m of aeolian sand burying at least 1.5 m of fluvial sediment (Fig. 8D). The two basal fluvial units (1 and 2) are fining upward sequences of sands, granules, and gravels (Facies 1) capped by a paleosol. Quartz grains from the upper fluvial unit (2) yielded an OSL age of 7915 ± 360 yr (BG4903), marking a time of aggradation. Overlying the fluvial sand is a tripartite aeolian sequence with each unit (3–5) fining upward (Facies 3), with a buried cambic soil horizon demarking the upper contact of each unit. A series of five OSL ages from Units 3, 4, and 5 (Fig. 8D) indicate sand sheet deposition at ca. 6.5, 4.3 to 3.9, and 3.1 to 0.5 ka, respectively. The uppermost, 5-m-thick unit (6), which encompasses dunes expressed at the surface, is a well-sorted, medium to fine sand, mostly massive Facies 4–type sediment. Quartz grains from the base, midway, and near the top of Unit 6 returned OSL ages of 515 ± 20 yr (BG4899), 420 ± 40 yr (BG4898), and 395 ± 20 yr (BG4897), respectively, indicating near- continuous aeolian deposition at this site between ca. 520 and 350 yr ago.
DISCUSSION
Oldest ca. 85 to 65 ka fluvial and aeolian depositional record of the western Red River
The oldest recognized fluvial sediments are revealed in cores RRCOR16-1, RR18-OK1, and RR18-OK2, which returned OSL ages from 85 to 70 ka (Fig. 9). These fluvial sediments fine upward from Facies 1 to 2, with the top of the aggradational limit (falling stage) demarked by a buried soil or truncated by emplacement of younger fluvial gravels ca. 26 ka (Fig. 8C). Thus, there was net fluvial aggradation of at least 8 m between ca. 80 and 60 ka, as shown in cores RR18-OK1 and RR18-OK2 (Fig. 8C). However, the elevation of the paleo-surface revealed by Geoprobe cores RRCOR16-1 and RR18-OK1 at 7 to 8 m above RRL is a closer estimate of the terrace height (Fig. 10). The subsequent burial of the terrace by 5 to 7 m of aeolian sediments in the past 60 ka elevated the land surface in places, yielding an apparent height of 12 to 15 m for this terrace in places. We informally labeled this older remnant terrace at 5 to 8 m above the Red River and associated deposits that are ca. 85 to 65 ka old as “Vernon” for a nearby town (Figs. 1, 10, and 11).

Figure 9. Frequency distribution of optically stimulated luminescence (OSL) ages for aeolian and fluvial sediments for western Red River study area.

Figure 10. Inferred changes in Red River water level relative to current elevation in the past 80 ka. OSL, optically stimulated luminescence.
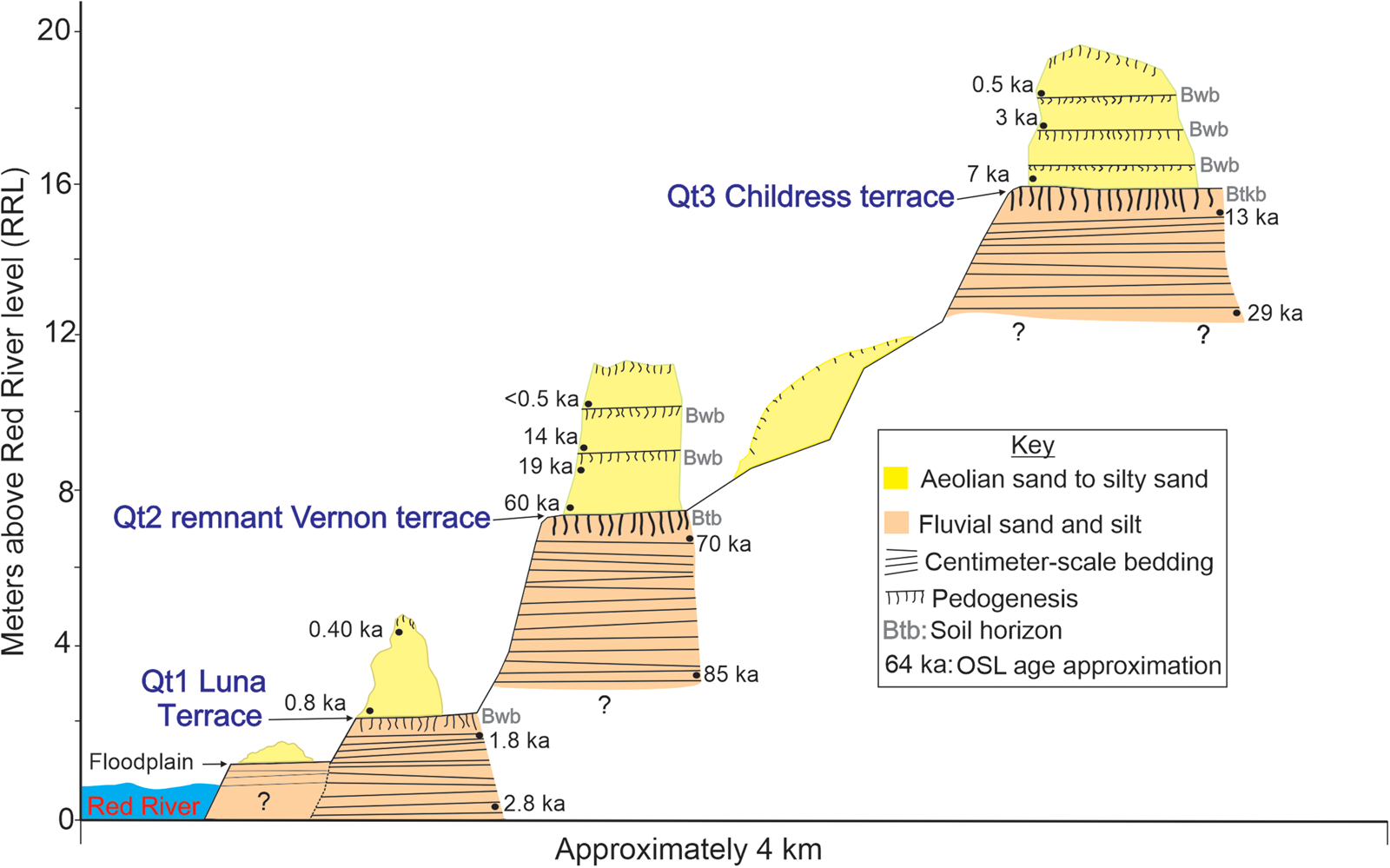
Figure 11. Diagrammatic cross section of western Red River terraces and fluvial and aeolian deposits with optically stimulated luminescence (OSL) age control.
Other fluvial deposits and river terraces have been identified in central and southern Texas that yield luminescence ages of ca. 80 to 65 ka consistent with the 7 to 8 m above RRL, buried Vernon surface. These fluvial deposits may be time equivalent with late Beaumont deposits along the Texas Coastal Plain emplaced during the transition from MIS 5 into cooling conditions in MIS 4 (Blum and Aslan, Reference Blum and Aslan2006). Similar OSL ages (ca. 72 to 64 ka) were reported for quartz grains from alluvial terrace (T2) sediments that host mammoth and other faunal remains at ~15 to 16 m above the Brazos River at the Waco Mammoth National Monument; and with abandonment of the terrace surface after ca. 52 ka (Nordt et al., Reference Nordt, Bongino, Forman, Esker and Benedict2015; Taormina et al., Reference Taormina, Nordt and Bateman2022). Another possible correlative deposit is an aggradational and truncated terrace (Q5) identified between 5 and 10 m above Owl Creek, a tributary to the Brazos River, in central Texas with bracketing OSL ages of ca. 75 and 44 ka (Meier et al., Reference Meier, Nordt, Forman and Driese2013). These surfaces may also be penecontemporaneous with the late Prairie Complex of the lower to middle Mississippi River valley, a fluvial deposit that reflects mesic conditions, shoreline progradation, and subsequent degradation with the fall in global sea level during the MIS 5 to MIS 4 transition (Dorale et al., Reference Dorale, Onac, Fornos, Gines, Gines, Tuccimei and Peate2010), possibly associated with late fluvial incision (Shen et al., Reference Shen, Törnqvist, Autin, Mateo, Straub and Mauz2012). Also, this oldest recognized fluvial deposit for the Red River appears to be time equivalent (80 to 60 ka) with the transition between the Beaumont and the Deweyville Formations, reflecting a period of pronounced incision post-Beaumont time, and initial aggradation from Deweyville surfaces along the Texas coast and associated inland fluvial systems (Blum and Aslan, Reference Blum and Aslan2006).
The oldest identified aeolian deposit is from core RR18-OK1 (Facies 3), as a <1-m-thick sandy silt (Facies 2) that yielded an OSL age of 60,210 ± 3010 yr BP (BG4960) (Figs. 8A and 9). This remnant, massive aeolian unit buries a truncated, well-developed buried soil (Btk horizon) formed in a fluvial deposit, with an OSL age of 72.2 ka (Fig. 8A), indicating that this buried soil has a maximum age span of ca. 12 ka, with terrace abandonment after 67 ka. In turn, this aeolian deposit was eroded with the deposition of the overlying aeolian sand ca. 37 ka. This period of aeolian deposition ca. 60 to 35 ka is consistent with a pronounced aeolian depositional phase in the Monahans dune field in west Texas burying a prominent paleosol and is associated with increased regional aridity (Forman et al., Reference Forman, Tew-Todd, Mayhack, Wiest and Money2022).
Little is known about the paleoenvironmental variability before the LGM in midcontinental North America, reflecting uncertainty about ice-sheet extent and dynamics (Dalton et al., Reference Dalton, Stokes and Batchelor2022). Sea level at ca. 80 ka during MIS 5a is constrained at or near levels similar to those today, though potentially short-lived for 5 to 10 ka (Dorale et al., Reference Dorale, Onac, Fornos, Gines, Gines, Tuccimei and Peate2010), with nearly full deglaciation of the North American continent (Gowan et al., Reference Gowan, Zhang, Khosravi, Rovere, Stocchi, Hughes, Gyllencreutz, Mangerud, Svendsen and Lohmann2021; Dalton et al., Reference Dalton, Stokes and Batchelor2022). Speleothem records from Crevice Cave, Missouri, show a series of short, positive oxygen isotope oscillations at ca. 74, 71, and 64 ka, reflecting 2°C to 3°C cooling events every 3 to 7 ka, with relative warming for intervening periods (Dorale et al., Reference Dorale, Edwards, Ito and Gonzalez1998). Associated carbon isotopes and pollen indicate an increasing dominance of grassland with high C4 biomass, an indication of drying (Dorale et al., Reference Dorale, Edwards, Ito and Gonzalez1998; Baker et al., Reference Baker, Bettis, Mandel, Dorale and Fredlund2009). Thus, the formation of the Vernon terrace between ca. 79 and 66 ka may be associated with repeated wet periods increasing runoff and subsequent dry conditions, and changing vegetation cover, all of which may have provided enhanced clastic inputs for net aggradation. Final downcutting and surface abandonment between 66 and 50 ka may be associated with regional aridity and cooling of North America and displacement of zonal circulation as the Laurentide Ice Sheet advanced into the midcontinent (Schmidt and Hertzberg, Reference Schmidt and Hertzberg2011; Dalton et al., Reference Dalton, Stokes and Batchelor2022).
LGM fluvial and aeolian depositional complex
The highest fluvial sediments identified in cores RRCOR16-3 and RRCOR18-2 are 15 to 20 m above the current channel and yielded associated OSL ages from the LGM to the earliest Holocene, ca. 30 to 12 ka (Figs. 7 and 8B). Similar high-elevation fluvial terraces (>5 m above the current channel) with associated ages between 25 and 12 ka have been identified along the Brazos (Waters and Nordt, Reference Waters and Nordt1995; Taormina et al., Reference Taormina, Nordt and Bateman2022) and the Pedernales Rivers (Blum and Valastro, Reference Blum and Valastro1994). This period of fluvial aggradation is associated with a regional positive water balance as indicated by the presence of paleo-Lake King in far western Texas (Wilkins and Currey, Reference Wilkins and Currey1997), other pluvial lakes in New Mexico (Allen, Reference Allen, Lucas, Morgan and Zeigler2005), and associated climate modeling (Lora and Ibarra, Reference Lora and Ibarra2019). Wetter conditions, particularly in winter, are associated with maximum glaciation of North America, with several proxy records indicating the periodic strengthening of zonal precipitation in the southwestern United States (Bartlein et al., Reference Bartlein, Harrison, Brewer, Connor, Davis, Gajewski and Guiot2011; Bhattacharya et al., Reference Bhattacharya, Tierney, Addison and Murray2018; Izumi et al., Reference Izumi, Valdes, Ivanovic and Gregoire2022), which would enhance sediment delivery into catchments during a season with low vegetation cover, resulting in pronounced aggradation (e.g., Waters et al., Reference Waters, Keene, Prewitt, Everett, Laughlin and Stafford2021).
Three cores—RR16-1, RR16-3, and RR18-OK1—show modest aeolian deposition during the LGM, ca. 25 to 13 ka (Figs. 7 and 8). These aeolian deposits are massive, moderately well-sorted, cover sands to loams, ≤1 m thick. In core RR18-OK1, the thin Unit 6 that yielded the basal OSL age of 14.3 ka is capped by a cambic horizon demarcating the end of the LGM (Fig. 8A).
Of note, the highest preserved Childress terrace at 15 to 20 m, with OSL ages of ca. 30 to 14 ka old, is not the oldest identified fluvial deposit. The oldest preserved fluvial sediment is associated with the Vernon terrace, 85 to 70 ka old, and often occurs beneath 3 to 4 m thickness of aeolian sediments, with a terrace top at 7 to 8 m, a lower elevation of the Childress terrace. The Vernon terrace is unpaired and reflects greater lateral migration than downcutting in the past 70 ka (Bierman and Montgomery, Reference Bierman and Montgomery2014, pp. 235–237) and preservation beneath >3-m-thick aeolian and fluvial deposits. Similar unpaired terraces and terrace remnants have been found for terraces and strath surfaces along the Colorado Front Range, reflecting complex channel dynamics with multiple reoccupations of river levels, variable channel migration, and erosion during the late Pleistocene (e.g., Dühnforth et al., Reference Dühnforth, Anderson, Ward and Blum2012; Foster et al., Reference Foster, Anderson, Gray and Mahan2017).
Holocene fluvial degradation and aggradation and aeolian deposition
There is an absence of recognized fluvial deposits from the Early to Middle Holocene, ca. 17.0 to 7.0 ka (Fig. 9). The oldest identified Holocene fluvial deposit yielded an OSL age of 7015 ± 360 yr (BG4903) from core RR18-OK7 (Fig. 8), proximal to the Red River, at about 4 m below the current river elevation. Though limited, these observations indicate that much of the early Holocene may have been a period of net fluvial degradation of ~20 m, from a height of 14 to 16 m above the current Red River at ca. 13 ka to at least 4 m below the channel by 7 to 6 ka (Fig. 10). However, after ca. 7 ka at the RR18-OK7 core site, aggradation or channel abandonment occurred, as indicated by basal fluvial sediments (Facies 2) capped by buried soils. In turn, overlying fluvial Unit 2 are >4 m thickness of sand sheet sediments (Facies 3) deposited between 6.5 and 0.5 ka (Fig. 8D). The deposition of this aeolian sand sheet occurred episodically at ca. 6.5 to ~4.6 ka, 4.3 to ~3.8 ka, and 3.2 to 0.5 ka, with OSL ages indicating that the bounding, well-drained, cambic buried soils reflect hiatuses of <2000 yr (Fig. 8D).
Fluvial aggradation continued into the Late Holocene from 4.0 to 1.5 ka, as shown in core RR18-OK3. This succession from channel gravels to overbank silts and sands (Facies 1 to 2) with a capping buried soil (Fig. 8C) indicates an aggradational limit and subsequent downcutting and/or abandonment of this fluvial surface (Fig. 11). Unconformably overlying these fluvial sediments is a <10-m-thick sequence of aeolian sand deposited between 600 and 300 yr ago. This Qt1 surface, informally named the “Luna” terrace, occurs 1 to 3 m above RRL and formed between ca. 1500 and 700 yr ago. The lowest and youngest recognized fluvial deposits at the Mike Site (Fig. 2A), are inset into the Luna terrace at 1.5 m RRL and yield OSL ages from 150 to 30 yr old (Fig. 6B), which shows nineteenth- and twentieth-century fluvial accretion and later incision. In turn, the Hollis Site, also at the current cutbank of the Red River (Fig. 2A), shows the association of aeolian of sand sheet deposited ca. 195 to 10 yr ago (Fig. 6C), sourced from the immediately adjacent floodplain surface, as inferred for earlier aeolian deposits at ca. 500 to 400 yr ago.
Little Ice Age, 500 to 400 years ago, source-border dune fields
A compelling result is the latest and most pronounced phase(s) of aeolian deposition occurred between 550 and 400 yr ago at most study sites (Figs. 6–8). This phase is recognized in cores and sections as a 1.7- to 2.0-m-thick deposit on the highest surfaces distal (6.5 km) from the Red River and thickens exponentially to 7 to 5 m at sites <2 km perpendicular distance from the river (Fig. 12), indicating a riverine source for this aeolian sediment. The exponential decrease in aeolian sand thickness that yielded OSL ages between 550 and 400 yr is similar to the decrease in Peoria loess thickness from bluff edges in the Mississippi and Missouri valleys proximal to this aeolian sediment source (Rodbell et al., Reference Rodbell, Forman, Pierson and Lynn1997; Muhs et al., Reference Muhs, Bettis, Aleinikoff, McGeehin, Beann, Skipp, Marshall, Roberts, Johnson and Benton2008).

Figure 12. Thickness of aeolian sediments dated between 550 and 300 yr ago in reference to perpendicular distance to the Red River. The thickness of this sedimentary unit thins exponentially from the River Red source onto adjacent terraces.
Aeolian deposition in the past 500 yr shows two potential subphases at its thickest stratigraphic occurrence in cores RR18-OK2 and RR18-OK3, and identified traceable units in the Pivot A and B sections with initial aeolian deposition occurred at ca. 550 to 450 yr BP. A later subphase of aeolian deposition spanned from 420 to 380 yr ago and was separated from the earlier phase by a distinct cambic buried soil. The apparent sedimentation rate of 11 to 4 cm/yr associated with these phases of aeolian deposition are similar in magnitude to other source-border aeolian systems associated with coastal settings (e.g., Maun, Reference Maun2009, p. 96; Han et al., Reference Han, Kim, Yang, Lim and Yi2021), further underscoring a proximal riverine source for aeolian sediments.
The latest phase of aeolian deposition occurs between AD 1590 and AD 1620 and appears to coincide with a comparatively wet period in the central United States associated with Little Ice Age cooling in the Northern Hemisphere (Cook et al., Reference Cook, Cook, Smerdon, Seager, Williams, Coats, Stahle and Diaz2016). This period of aeolian deposition may also partially overlap with or closely follow, considering errors in OSL ages, a sixteenth-century megadrought between AD 1570 and AD 1590 (Stahle et al., Reference Stahle, Fye, Cook and Griffin2007; Cook et al., Reference Cook, Williams, Smerdon, Palmer, Cook, Stahle and Coats2018). Landscape denudation associated with a regional drought can yield pronounced sediment delivery into adjacent fluvial systems, particularly if followed by excess runoff with pluvial conditions, leading to aggradation (Hall, 1990) and enhanced fluvial sediment supply for aeolian transport in channel proximal dunes. A pronounced dune accretion phase 300 to 500 yr ago has also been identified along the Arkansas River valley in western and eastern Kansas (Arbogast, Reference Arbogast1996; Forman et al., Reference Forman, Marin, Gomez and Pierson2008; Halfen, Reference Halfen2012). The closest tree ring–based Palmer Drought Severity Index (PDSI) record is near the headwaters of the Red River in the Sangre de Cristo Mountains and shows a pronounced wet interval for the late sixteenth and seventeenth centuries (Fig. 13). This record shows wet years with PDSI values up to +4 often separated by years with PDSI values ranging from 0.5 to −2.0. These inferred wet years may be coincident with flood years inferred for the middle Mississippi River valley from core records for an oxbow lake (Munoz et al., Reference Munoz, Gruley, Massie, Fike, Schroeder and Williams2015, Reference Munoz, Giosan, Therrell, Remo, Shen, Sullivan, Wiman, O'Donnell and Donnelly2018; Fig. 13). Flood and/or high discharge events would provide added sediment supply across the Red River valley that would become available with waning flood stage and subsequent drying (e.g., Sankey et al., Reference Sankey, Caster, Kasprak and East2018). Thus, aeolian deposition in the late sixteenth and early seventeenth centuries is associated with wetter conditions providing new sources of sediment for river-proximal aeolian deposition on adjacent terraces. Subsequent reactivation of aeolian systems 200 and 90 yr ago may be associated with the Civil War and 1930s Dust Bowl Droughts with heightened anthropogenic activity (Woodhouse et al., Reference Woodhouse, Lukas and Brown2002; Bolles et al., Reference Bolles, Sweeney and Forman2019).

Figure 13. Comparison of proxy environmental records for the past 1000 yr. (A) Mississippi River paleoflood record from Horseshoe Lake, near Cahokia Site, IL (Munoz et al., Reference Munoz, Gruley, Massie, Fike, Schroeder and Williams2015). (B) June, July, and August Palmer Drought Severity Index for headwaters of the Red River in the Sangre de Cristo Mountains, NM (Cook et al., Reference Cook, Seager, Heim, Vose, Herweijer and Woodhouse2010). (C) Distribution of optically stimulated luminescence (OSL) ages on aeolian sand deposits on fluvial terraces, western Red River (this study). (D) Distribution of OSL ages for aeolian sand deposits associated with Cimarron River, OK (Lepper and Scott, Reference Lepper and Scott2005). (E) Distribution of OSL ages for aeolian sand deposits associated with Arkansas River dunes, southeastern Kansas (Forman et al., Reference Forman, Marin, Gomez and Pierson2008).
CONCLUSION
Geomorphic mapping identified three distinct terraces levels up to 15 to 20 m above the Red River, often covered by 2 to 8 m of aeolian sand forming presently stabilized source-border dune fields (Fig. 11). Pedostratigraphic, granulometric, carbonate content analysis in tandem with OSL-SAR dating of quartz grains from four stratigraphic sections and six Geoprobe cores from terraces covered by aeolian deposits provide new insights into the relation between fluvial dynamics and aeolian depositional phases (Figs. 11 and 13). The oldest fluvial landform, ca. 80 ka old, covered by 3 to 6 m of aeolian sand, is informally named the Vernon terrace, which occurs at 5 to 8 m above the current Red River. The Vernon terrace occurs as a remnant and may be associated with hydrologic variability during the transition from interglacial to glacial conditions ca. 85 to 65 ka, with enhanced clastic input for net aggradation. This surface was abandoned and incised sometime between 70 and 60 ka possibly in association with inferred regional aridity and cooling with the advance of the Laurentide Ice Sheet into the midcontinent of North America (Dorale et al., Reference Dorale, Edwards, Ito and Gonzalez1998; Dalton et al., Reference Dalton, Stokes and Batchelor2022).
The highest fluvial sediments identified are 15 to 20 m above the current Red River and yielded OSL ages of between 30 and 13 ka and capped in places by 4 to 5 m thickness of Holocene aeolian sediments (Fig. 11). This surface has been informally named the Childress terrace and is associated with an extended ca. 15 ka period with net fluvial aggradation. This period appears coincident with regional positive water balance with highstands of pluvial lakes in west Texas and adjacent New Mexico (Wilkins and Currey, 1997). Aeolian deposition appears to be sparse during the LGM on Red River terraces, with the uncommon occurrence of 1 to 2 m of pedogenically modified cover loam and sand.
There is a noticeable absence of fluvial deposits with OSL ages between 12.0 and 7.0 ka (Fig. 9). The oldest identified Holocene fluvial deposit is 7 ka old and occurs ~4 m below the current river channel. Thus, the Early to Middle Holocene was a time of net fluvial degradation of 15 to 20 m from the Childress terrace (Figs. 10 and 11), associated potentially with regional aridity with lowering of groundwater level. However, sometime between 7 and 1 ka, there was net aggradation to about 2 m RRL, forming the Luna terrace surface, with abandonment sometime between 1.5 and 0.7 ka (Fig. 11). Aeolian sediments, 4 to 10.5 m thick and spanning from 6.5 to 0.2 ka, were deposited on terrace surfaces (Fig. 11), with thickness dependent on the proximity to the riverine source (Fig. 12).
A compelling result is that dune accretion across terraces occurred between 500 and 300 yr ago with an apparent sedimentation rate of 11 to 3 cm/yr, similar in magnitude to coastal aeolian systems (e.g., Maun, Reference Maun2009, p. 96; Han et al., Reference Han, Kim, Yang, Lim and Yi2021), thus underscoring a proximal riverine source for the Red River dune fields. A pronounced dune accretion phase 300 to 500 yr ago has also been identified along the Arkansas River valley in western and eastern Kansas (Arbogast, Reference Arbogast1996; Forman et al., Reference Forman, Marin, Gomez and Pierson2008; Halfen, Reference Halfen2012; Halfen et al., Reference Halfen, Johnson, Hanson, Woodburn, Young and Ludvigson2012). This sixteenth- and seventeenth-century phase of dune building occurred during a comparatively cool and wet period in the central United States, the Little Ice Age, and is coincident with major flood intervals in the middle Mississippi River (Munoz et al., Reference Munoz, Gruley, Massie, Fike, Schroeder and Williams2015, Reference Munoz, Giosan, Therrell, Remo, Shen, Sullivan, Wiman, O'Donnell and Donnelly2018; Fig. 13). Sediment availability and sources for aeolian transport were heightened post high river discharge and flood events exposing sand and silt-rich fluvial surfaces as sources to accrete dune systems on river proximal terraces. Fluvial source-border dune fields adjacent to the Red River and other rivers on the SGP may reflect hydrologic variability with flooding rather than the common and counterinterpretation of drought or megadrought conditions (Forman et al., Reference Forman, Oglesby, Markgraf and Stafford1995, Reference Forman, Oglesby and Webb2001, Reference Forman, Marin, Gomez and Pierson2008; Hanson et al., Reference Hanson, Arbogast, Johnson, Joeckel and Young2010; Halfen and Johnson, Reference Halfen and Johnson2013; Halfen et al., Reference Halfen, Lancaster and Wolfe2016).
Supplementary Material
The supplementary material for this article can be found at https://doi.org/10.1017/qua.2023.15.
Acknowledgments
This research was supported initially through grants from the National Science Foundation (award GSS-1660230) and the National Geographic Society (no. 9990-16). Access to study sites was generously provided by local landowners on both sides of the state boundary. Discussions with M. Waters, who freely shared his knowledge, were enlightening. Comments from two anonymous reviewers and the editors were appreciated and improved the presentation. Each study reveals partially, and all shortcomings are solely the responsibility of the authors.