Introduction
The last decade (2010–2020) has been marked by a new wave of interest in clays and clay minerals. Many applications have been found, in fields ranging from adsorption and water remediation to catalysis and medicine, and these are due to the natural abundance and morphological and structural diversity of clay minerals. Among these minerals, hydrosilicates are an interesting group which are able to scroll spontaneously: Al2SiO3(OH)4 imogolite (Paineau et al. Reference Paineau, Krapf, Amara, Matskova, Dozov, Rouzière, Thill, Launois and Davidson2016; Shafia et al. Reference Shafia, Esposito, Armandi, Manzoli, Garrone and Bonelli2016; Arancibia-Miranda et al. Reference Arancibia-Miranda, Escudey, Ramírez, González, van Duin and Kiwi2017; Picot et al. Reference Picot, Liao, Barruet, Gobeaux, Coradin and Thill2018), Mg3Si2O5(OH)4 chrysotile (Bloise et al. Reference Bloise, Belluso, Fornero, Rinaudo, Barrese and Capella2010; Korytkova et al. Reference Korytkova, Brovkin, Maslennikova, Pivovarova and Drozdova2011; Lafay et al. Reference Lafay, Fernandez-Martinez, Montes-Hernandez, Auzende and Poulain2016; Krasilin et al. Reference Krasilin, Nevedomsky and Gusarov2017; Maslennikova and Gatina Reference Maslennikova and Gatina2017; López-Salinas et al. Reference López-Salinas, Toledo-Antonio, Manríquez, Sánchez-Cantú, Cruz Ramos and Hernández-Cortez2019; Bian and Kawi Reference Bian and Kawi2020), and Al2Si2O5(OH)4 halloysite (Cataldo et al. Reference Cataldo, Lazzara, Massaro, Muratore, Pettignano and Riela2018; Krasilin et al. Reference Krasilin, Danilovich, Yudina, Bruyere, Ghanbaja and Ivanov2019b; Lvov et al. Reference Lvov, Panchal, Fu, Fakhrullin, Kryuchkova, Batasheva, Stavitskaya, Glotov and Vinokurov2019). Their crystal structures combine two covalently bonded sheets of metal-oxygen octahedra and silicon-oxygen tetrahedra (joined in a network or separated as in the case of imogolite). Substantial differences in the size of the sheets and in terms of their structure give rise to a bending momentum, which transforms the layers into single-walled or multi-walled tubular, conical (Bloise et al. Reference Bloise, Belluso, Barrese, Miriello and Apollaro2009b; Krasilin et al. Reference Krasilin, Suprun, Nevedomsky and Gusarov2015), or even spheroidal (Berthonneau et al. Reference Berthonneau, Grauby, Jeannin, Chaudanson, Joussein and Baronnet2015; Cravero et al. Reference Cravero, Fernández, Marfil, Sánchez, Maiza and Martínez2016; Thill et al. Reference Thill, Picot and Belloni2017; Du et al. Reference Du, Thill, Yuan, Wang, Liu, Gobeaux, Deng and Song2020) particles. This intriguing structural feature has led to theoretical research based on the density functional theory method (Guimarães et al. Reference Guimarães, Enyashin, Seifert and Duarte2010; Lourenço et al. Reference Lourenço, de Oliveira, Oliveira, Guimarães and Duarte2012; da Silva et al. Reference da Silva, dos Santos, Lourenço, Gouvea and Duarte2015; Poli et al. Reference Poli, Elliott, Hine, Mostofi and Teobaldi2015, Reference Poli, Elliott, Ratcliff, Andrinopoulos, Dziedzic, Hine, Mostofi, Skylaris, Haynes and Teobaldi2016; Demichelis et al. Reference Demichelis, De La Pierre, Mookherjee, Zicovich-Wilson and Orlando2016), molecular dynamics (Prishchenko et al. Reference Prishchenko, Zenkov, Mazurenko, Fakhrullin, Lvov and Mazurenko2018; Gonzalez et al. Reference González, Valencia, Rogan, Valdivia, Sofo, Kiwi and Munoz2018), hydrodynamics (Chivilikhin et al. Reference Chivilikhin, Popov and Gusarov2007), and phenomenological modeling (Singh Reference Singh1996; Perbost et al. Reference Perbost, Amouric and Olives2003; Chivilikhin et al. Reference Chivilikhin, Popov, Svitenkov, Chivilikhin and Gusarov2009; Thill et al. Reference Thill, Guiose, Bacia-Verloop, Geertsen and Belloni2012; Krasilin and Gusarov Reference Krasilin and Gusarov2014). Most tubular minerals can be synthesized successfully in the laboratory using soft chemical and hydrothermal techniques; this is not true of halloysite.
Few reports about the hydrothermal synthesis of tubular halloysite can be found in the literature (White et al. Reference White, Bavykin and Walsh2012) and those mention the very small yield of nanotubes and nanoscrolls in comparison to platy particles. To date, the principal approach to producing tubular halloysite has been exfoliation of its platy analog, kaolinite (Kuroda et al. Reference Kuroda, Ito, Itabashi and Kuroda2011; Liu et al. Reference Liu, Li and Cheng2016; Makó et al. Reference Makó, Kovács, Antal and Kristóf2017; Zsirka et al. Reference Zsirka, Táborosi, Szabó, Szilágyi, Horváth, Juzsakova, Fertig and Kristóf2017; Li et al. Reference Li, Wang, Liu and Komarneni2019), and most of the application-oriented studies have involved mined halloysite (Cavallaro et al. Reference Cavallaro, Danilushkina, Evtugyn, Lazzara, Milioto, Parisi, Rozhina and Fakhrullin2017; Ma et al. Reference Ma, Wu, Higaki and Takahara2018; Goda et al. Reference Goda, Gab-Allah, Singu and Yoon2019). Theoretical research on the halloysite layer (Guimarães et al. Reference Guimarães, Enyashin, Seifert and Duarte2010; Ferrante et al. Reference Ferrante, Armata and Lazzara2015; Prishchenko et al. Reference Prishchenko, Zenkov, Mazurenko, Fakhrullin, Lvov and Mazurenko2018) has confirmed its significant scrolling potential. Chrysotile layers, which have a very similar structure, scroll with ease in hydrothermal conditions.
In previous studies, an energy model of multi-walled nanoscroll formation was developed by Krasilin and Gusarov (Reference Krasilin and Gusarov2015, Reference Krasilin and Gusarov2016). The model explained (Mg,Ni)3Si2O5(OH)4 diameter and length distributions of the nanoscrolls (Krasilin et al. Reference Krasilin, Nevedomsky and Gusarov2017), as well as the increase in the amount of conical nanoscrolls (Krasilin and Gusarov Reference Krasilin and Gusarov2017). For the present study, the model was applied to a description of the energy effect of multi-walled plate formation of chrysotile and halloysite followed by comparison with the energy effect of scrolling of corresponding layers. The main purpose was to unearth hindrances which prevent halloysite particles from spontaneous scrolling in artificial hydrothermal conditions.
THEORY
Energy Effect of Scrolling
The energy model consists of a layer composed of two sheets of different size and structure (Fig. 1a). These differences initiate the scrolling. The energy effect of scrolling is written as follows:

where ν is the amount of substance,
and
are strain energies of the scrolled (@) and flat (/) layers, Σ@ and Σ/ are surface energies of the scrolled and flat layers, and
is the adhesion energy of a multi-walled scroll.
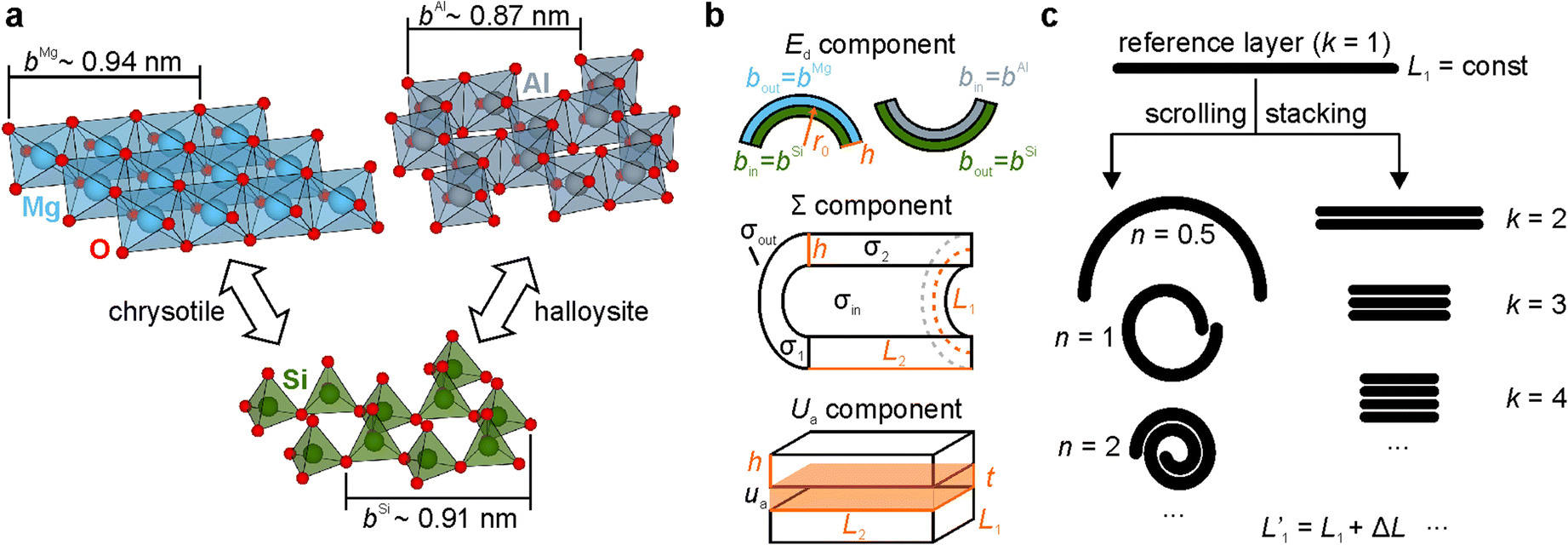
Fig. 1. Calculation concepts. a Covalent bonding of two different sheets yielded chrysotile and halloysite layers. Sheet structures were adopted from Saalfeld and Wedde (Reference Saalfeld and Wedde1974), Desgranges et al. (Reference Desgranges, Calvarin and Chevrier1996), Churakov et al. (Reference Churakov, Iannuzzi and Parrinello2004), and Roveri et al. (Reference Roveri, Falini, Foresti, Fracasso, Lesci and Sabatino2006) using VESTA software (Momma and Izumi Reference Momma and Izumi2011). b Structural parameters of the layers. c Scrolling and stacking of layers
Assuming that a nanoscroll cross-section can be described by the Archimedean spiral,
is expressed through integration in polar coordinates:

where D s is bending stiffness, L 2 is the length of the scroll (i.e. length of the cylinder), n is the number of layers within the wall, r(φ) is the current radius, r 0 is the radius of the mechanically unstressed layer, and f is the spiral constant. The part under the square root is the length of the integration element, which yields the spiral length after integration.
If the layer can be described using the continuous mechanics approach (Landau et al. Reference Landau, Pitaevskii, Kosevich and Lifshitz1986), the bending stiffness can be shown as:

where Y is Young’s modulus of the layer, h is the thickness of the layer, and μ is the Poisson ratio.
The Archimedean spiral attributes are:

where r in is the inner (initial) radius of the scroll and t is the interlayer spacing.
The r 0 value depends on size of the sheets (Fig. 1b):

where b out and b in are the size of the outer and inner sheets, respectively, i.e. lattice constants.
The
value of Mg3Si2O5(OH)4 chrysotile is well known (Cressey and Whittaker Reference Cressey and Whittaker1993; Demichelis et al. Reference Demichelis, De La Pierre, Mookherjee, Zicovich-Wilson and Orlando2016), and one could estimate
for some scrolls of another chemical composition. Assuming constant layer thickness, the size of the tetrahedral sheet and the direction of the new scrolling
value is:

where b ∗ is proportional to the lattice constant of metal hydroxide and b Mg is proportional to the lattice constant of Mg(OH)2. In cases where the direction of scrolling is opposite to that of chrysotile:

Note that b Mg and b ∗ must correspond with each other in terms of an equal number of polyhedra (Fig. 1a).
The strain energy of the flat layer is:

where L 1 is the length of the flat layer along the direction of scrolling, which is equal to spiral length.
The surface energy of a scroll is the sum of all surface areas multiplied by specific surface energy values:

where σout, σin, σ1, and σ2 are the specific surface energies of the outer, inner, and the two-edge surfaces, respectively.
The lengths
and
of the spirals formed by outer and inner surfaces are determined as follows:

In the case of the flat layer, Eq. 9 is simplified to:

The last component of Eq. 1, the adhesion energy, can be estimated using the same principle if n > 1:

where u a is the specific adhesion energy.
Specific surface and adhesion energy values are assumed to be independent of the radius of curvature. Usually the size effects must be taken into account if the radius is <1–2 nm (Tolman Reference Tolman1949; Dumitrică et al. Reference Dumitrică, Landis and Yakobson2002; Vollath et al. Reference Vollath, Fischer and Holec2018; Holec et al. Reference Holec, Dumitraschkewitz, Vollath and Fischer2020).
Energy Effect of Multi-layered Plate Formation
A single flat layer of length L 1 can be divided into k equal parts (Fig. 1c), which are then stacked together to form a multi-layered rectangular plate. This procedure increases the surface energy of the plate due to the formation of new edge surfaces. On the other hand, the stacking of layers decreases their surface energy due to adhesion. As for strain energy, partition of the layer does not influence its value. Thus, the energy effect of multi-layered plate formation is:

The amount of substance, which is assumed to be constant during the scrolling process, can be calculated as follows:

where ρ is the density of the layer and M is the molar mass.
The surface energy of the plate is:

where k = 1, 2, 3…
The adhesion energy when k > 1 is:

The number of k layers stacked together in a plate is an integer equal to the number of layers, n. For the sake of comparison, the calculation results are represented in the same plots, considering k = n where needed.
The specific adhesion energy, u a, is calculated as the sum of hydrogen bond energies per unit area:

where CK is a proportionality constant, NA is Avogadro’s number, N H is the number of hydrogen bonds between adjacent hydrosilicate layers within one cell, a and b are lattice constants, and CW = 1.2 ∙ 1010 (if t is expressed in pm) is the fitting constant proposed by Wendler et al. (Reference Wendler, Thar, Zahn and Kirchner2010).
Determination of Model Parameters
Equations 1 and 13 are solved numerically in order to find the energy effect of either multi-walled scroll or plate formation in comparison to a single flat layer of the same mass (Fig. 1). In Table 1 are given the numerical values of a number of structural parameters of the model. Chrysotile and halloysite scrolling directions are opposite (Krasilin et al. Reference Krasilin, Danilovich, Yudina, Bruyere, Ghanbaja and Ivanov2019b), so in order to estimate the
value for halloysite, Eq. 7 is used, which yielded 3.3 nm. Remarkably, the same value was also obtained by solving an empirical equation proposed by Guimarães et al. (Reference Guimarães, Enyashin, Seifert and Duarte2010) as a fit of DFT calculations. Chrysotile and halloysite (7 Å modification) had almost equal layer thicknesses and interlayer spacings. Some previous theoretical and experimental results indicated that Young’s moduli of the nanoscrolls were also similar (Piperno et al. Reference Piperno, Kaplan-Ashiri, Cohen, Popovitz-Biro, Wagner, Tenne, Foresti, Lesci and Roveri2007; Guimarães et al. Reference Guimarães, Enyashin, Seifert and Duarte2010; Lourenço et al. Reference Lourenço, de Oliveira, Oliveira, Guimarães and Duarte2012; Lecouvet et al. Reference Lecouvet, Horion, D'Haese, Bailly and Nysten2013); a significant scatter of experimental values was found, however. The influence of Young’s modulus on the position and form of the multi-walled nanoscroll energy minimum can be found elsewhere (Krasilin and Gusarov Reference Krasilin and Gusarov2016).
Table 1. Structural parameters of the energy model for chrysotile and halloysite layers

athe value is the Mg(OH)2 brucite cell parameter multiplied by 3; b(0001) surface of Mg(OH)2 brucite; c(111) surface of α-SiO2 cristobalite; d(002) surface of Al(OH)3 gibbsite; esee the text for comments regarding the range.
In order to assign specific surface energy values, the data for corresponding metal hydroxides and silica surfaces are used. The value of 3 meV/Å2 (0.05 J/m2) for the (0001) Mg(OH)2 surface was used by Churakov et al. (Reference Churakov, Iannuzzi and Parrinello2004); in the opinion of those authors, that value might have been an underestimate. For Al(OH)3, the value of 0.22 J/m2 for the (002) surface was used, obtained by Fleming et al. (Reference Fleming, Rohl, Lee, Gale and Parkinson2000). The choice of the σ value for the silica sheet surface was complicated by the fact that no close analogue has been found among known crystal structures, so the value of 1.51 J/m2 for the (111) α-SiO2 surface (Shchipalov, Reference Shchipalov2000) is taken as a reasonable approximation.
Because of this uncertainty with edge surfaces, the model has difficulty distinguishing confidently between σ1 and σ2 numerical values, so the case of σ1 = σ2 = σe is considered. The edge surfaces are a mixture of metal hydroxide and silica surfaces, so whether they are hydroxylated or not is unclear. The calculation studied the scrolling process in the range of 0.5–3.0 J/m2 of σe values. This range was determined by two arguments. First, all of the above-mentioned reports (Fleming et al. Reference Fleming, Rohl, Lee, Gale and Parkinson2000; Shchipalov Reference Shchipalov2000; Churakov et al. Reference Churakov, Iannuzzi and Parrinello2004) claimed that σ values of the edge surfaces –
Mg(OH)2, (200) Al(OH)3, and, to some extent, (001) α-SiO2 – were at least twice as large as those of (0001), (002), and (111) surfaces, respectively. Second, surface dehydroxylation usually increased the specific surface energy; typical σ values of MgO and α-Al2O3 were 1.5 and 2.0 J/m2 (Mackrodt et al. Reference Mackrodt, Davey, Black and Docherty1987; Evarestov and Bandura Reference Evarestov and Bandura2004).
Finally, estimation of the u a value using Eq. 17 yielded ~0.11 J/m2 for both chrysotile (a = 0.530 nm, b = 0.922 nm) (Krasilin et al. Reference Krasilin, Nevedomsky and Gusarov2017) and halloysite (a = 0.513 nm, b = 0.879 nm) (Zhang et al. Reference Zhang, Lei, Yan, Wang, Xiao, Hao, Wang and Qiu2011) layers at 5–6 hydrogen bonds per cell.
By substituting the parameters from Table 1 into Eqs 1 and 13, the energy effects of multi-walled scroll or plate formation were evaluated. In the calculation, the spiral length L 1 varied from 10 nm to 2 μm with a 5 nm step, whereas length L 2 was fixed at 1 μm; in fact, any value could be assigned to L 2 as long as the assumption σ1 = σ2 = σe was acceptable. For each value of L 1, a local energy minimum was found by changing n with a 0.1 step in correlation with r in (or by changing k with a 1 step in the case of plate formation, see Fig. 1c). This set of points then formed the energy-effect curve represented in Fig. 2a,b.

Fig. 2. Preferred size parameters (inner and outer radii of curvature) and energy effect of scrolling (
) of a chrysotile and b halloysite layers. The position of the principal energy minimum is marked by a vertical line. Influence of Young’s modulus on c chrysotile and d halloysite preferred radii of curvature. Influence of the specific surface energy value of the octahedral sheet (σoct, which is equal to σout or σin) on e chrysotile and f halloysite preferred radii of curvature
The Young’s modulus and specific surface energy of the octahedral sheet (σout or σin) were varied in order to show their influence on the preferred size parameters of chrysotile and halloysite nanoscrolls. Meanwhile, one value was varied and the rest were fixed in correspondence with Table 1. The results are shown in Fig. 2c–f.
RESULTS AND DISCUSSION
Preferred Size Parameters of Chrysotile and Halloysite Nanoscrolls
During spiral-length increase, both chrysotile and halloysite layers demonstrated energy minima (Fig. 2a,b), which originated from an excess strain energy in the growing spiral. No discrepancy was observed between the inner and outer radii curves at small L 1, because curved layers with n ≤ 1 were more energy effective than multi-walled structures. Preferred size parameters for chrysotile nanoscrolls were: inner radius = 1.9 nm, outer radius = 6.6 nm, 7–8 layers; for halloysite they were: inner radius = 3.2 nm, outer radius = 17.5 nm, 21–22 layers. Surface-energy differences on the opposite side of the layer in the case of chrysotile favored scrolling, whereas in the case of the halloysite layer bending the layer, in the opposite direction was the tendency (Krasilin and Gusarov Reference Krasilin and Gusarov2014). That is why the energy minimum of the latter was shallower and wider than that of chrysotile. The position and form of energy minima did not depend on the σe value, because the corresponding terms in Eqs 9 and 11 were reduced by subtraction.
In contrast with previous modeling by Krasilin et al. (Reference Krasilin, Nevedomsky and Gusarov2017), some of the model parameters (in particular σout, σin, and u a) responsible for the surface and adhesion energy components were essentially changed. These changes did not affect the modeling results qualitatively, but they yielded a new quantitative estimate of the value of the energy effects.
Variation of the Young’s modulus value (Fig. 2c,d) and specific surface energy of the octahedral sheet (Fig. 2e,f) had opposing effects on the chrysotile and halloysite preferred radii of curvature. This was probably related to differences in the strain and surface-energy components: in the case of chrysotile layers these two directions are the same whereas in the case of halloysite layers the two directions are opposite. As a result, halloysite layers were more sensitive to parametric variation. Overestimation of the halloysite layer Young’s modulus could lead to discrepancies between calculated and experimental values of the radii of curvature. For example, Lvov and Abdullayev (Reference Lvov and Abdullayev2013) reported the halloysite nanotube inner radius as being in the 2.5–5 nm range and the outer radius being in the 25–50 nm range. Using a Young’s modulus value of 250 GPa instead of 300 GPa was sufficient to obtain the reported values.
Another possible reason for the discrepancy is the conditions of formation of the nanoscrolls; these include temperature, pressure, pH, presence of impurities, and other parameters. In the case of synthetic chrysotile and pecoraite (Ni-based structural analog), increases in temperature and pH yielded increases in the number of layers, outer diameter, and length (Korytkova et al. Reference Korytkova, Brovkin, Maslennikova, Pivovarova and Drozdova2011; Bloise et al. Reference Bloise, Belluso, Catalano, Barrese, Miriello and Apollaro2012; Krasilin et al. Reference Krasilin, Nevedomsky and Gusarov2017) because they caused significant oversaturation by the hydrothermal medium and intense precipitation of the substance on more available nanoscroll side surfaces. Replacement of 72 pm Mg, 53 pm Al, and 26 pm Si by other cations, e.g. by 69 pm Ni (Bloise et al. Reference Bloise, Belluso, Fornero, Rinaudo, Barrese and Capella2010; Krasilin et al. Reference Krasilin, Nevedomsky and Gusarov2017, Reference Krasilin, Khrapova, Nominé, Ghanbaja, Belmonte and Gusarov2019a), 61 pm Fe(II), 55 pm Fe(III) (Korytkova et al. Reference Korytkova, Pivovarova, Semenova, Drozdova, Povinich and Gusarov2007; Bloise et al. Reference Bloise, Belluso, Barrese, Miriello and Apollaro2009b), and 39 pm Ge (Perbost et al. Reference Perbost, Amouric and Olives2003; White et al. Reference White, Bavykin and Walsh2012), with respect to the energy model, would change the r 0 value (see Eqs 2 and 5) and, consequently, the preferred size parameters of the nanoscroll (ionic radii taken from Shannon Reference Shannon1976). In the case of chrysotile, these substitutions increased the r 0 value and the diameters of the particles. In the particular case of Ge substitution for Si in halloysite, the r 0 value decreased, imparting additional stimuli for the layer to scroll. The difference in ionic radii between these cations is rather large, however, and this led to formation of Ge-doped imogolite nanotubes at high Ge content, as observed by White et al. (Reference White, Bavykin and Walsh2012). The 42 pm Ti content in doped chrysotile nanoscrolls (Bloise et al. Reference Bloise, Barrese and Apollaro2009a; Maslennikova and Gatina Reference Maslennikova and Gatina2018) is limited for the same reason.
Scroll vs Plate
The energy effect of scrolling (
) and the energy effect of multi-layered plate formation (
) are compared in Fig. 3. According to Ogorodova et al. (Reference Ogorodova, Kiseleva, Korytkova and Gusarov2006), the enthalpies of formation of natural and synthetic chrysotile were 420 kJ/mol and 390 kJ/mol, respectively; so the calculated energy effect of scrolling was at least an order of magnitude weaker (Fig. 3). The comparatively small calculated energy effect of scrolling could explain some difficulties with the experimental observation of the heat effect of scrolling on the background heat effect caused by recrystallization of the starting components (Sharikov et al. Reference Sharikov, Korytkova and Gusarov2007).

Fig. 3. Energy effect of scrolling (
), formation of a multi-layered plate (
), and the difference curve
The specific surface energy of the edges made an essential contribution to
. Its decrease favored the initial layer partition and stacking with the formation of a multi-layered structure. As a result, the energy effect of plate formation grew because of the increased contribution of the adhesion energy component (Eq. 16). Due to the absence of the strain energy component in Eq. 13,
did not have a minimum, and this prevented determination of the preferred size parameters of that particle. This feature made the platy morphology of the layer less energy preferable than the scrolled morphology for almost the entire range of particle sizes. However, the halloysite layer showed a comparatively small energy gap between scroll and plate, and the latter can be even more preferable at small σe and small spiral length L 1 (particle size).
A closer look at the L 1 = 20..100 nm region is provided in Fig. 4. At the spiral length L 1 = 20 nm (length of a single layer) and with small specific surface energy at the edges, σe = 0.5 J/m2, the halloysite layer much preferred to form a two-layered 10 nm plate than to scroll, because the total effect of adhesion (Eq. 16) exceeded the effect of edge surface area increase (Eq. 15). The length L 1 = 30 nm was already sufficient for the halloysite layer to form a nanoscroll of n ≈ 1.5, and the energy curve was composed of two overlapping minima due to the contribution of adhesion energy. But this contribution was still insufficient to make the halloysite scroll geometry more energy preferable than the plate, unless the size of the layer reached some critical value, in this case 50 nm.

Fig. 4. Energy effect of scrolling (
) and formation of a multi-layered plate (
) vs. the number of layers (n, k) in the case of halloysite with σe = 0.5 J/m2. The points denoting the energy effect of plate formation are joined by a dashed line for the sake of clarity. The insets show the approximate geometry of the cross sections of the particles
If the L 1 value increase is considered to be nanoparticle growth during synthesis by adding a portion of substance to the system, then the calculation results shown in Fig. 4 highlight a substantial kinetic hindrance for a layer with a halloysite structure to form a nanoscroll at a given set of parameters. Initial precipitation of additional substance occurred on some of the particle surfaces proportionally to their area (Ivanov et al. Reference Ivanov, Fedorov, Baranchikov and Osiko2014), and this feature prevented the growth of one long layer. Later, when length L 1 reached 100 nm, for example, how could the halloysite plate that has already been formed turn into the more energy-preferred nanoscroll? Within the current approximation, only two options exist: full recrystallization of the plate, which would require the presence of additional nuclei in the system, or scrolling of the outer layer of the plate. The latter has been under consideration by Chivilikhin et al. (Reference Chivilikhin, Popov and Gusarov2007). Here, the actual size of the layer of the 5-layered plate was only 20 nm, and this was insufficient to form a scroll. Even if the plate were larger, the outer layer would need some external stimuli to overcome hydrogen bonding. Use of an intercalation agent that would weaken the adhesion would be such a stimulus (Kuroda et al. Reference Kuroda, Ito, Itabashi and Kuroda2011).
An important remark regarding the form of the real cross-section should be considered. The curling process could yield both nanoscrolls and nanotubes in some ratio, which, to date, is unknown for chrysotile and halloysite. On the contrary, single- and double-walled imogolites are known to exist as nanotubes, although Thill et al. (Reference Thill, Guiose, Bacia-Verloop, Geertsen and Belloni2012) reported that multi-walled nanoscrolls of Ge-doped imogolite are a possible exception. The model presented was constructed involving spiral forms of cross sections because otherwise one had to find the energy effect of nanotube formation by solving equations with sums instead of integrals (Belloni and Thill Reference Belloni, Thill, Yuan, Thill and Bergaya2016). Assuming that the main contribution is related to the joining of two edge surfaces, the energy effect of nanotube formation instead of a nanoscroll could be estimated as follows:

The value was inversely proportional to the spiral length, so for multi-walled particles the effect was expected to be negligible. In the case of the calculation represented in Fig. 4, this effect could be taken into account by some natural n values. If L 1 = 20 nm, the effect would be –5.5 kJ/mol. This would put a total energy effect at n = 1 down to –3 kJ/mol (Fig. 4). At L 1 equal to 30 and 50 nm the effects would be –3.7 and –2.2 kJ/mol, respectively. An addition of these values to the points at n = 1 and n = 2 did not change the conclusion about the energy preference of platy particles.
For real systems containing a large number of particles, other factors can govern their morphology. An ensemble of imogolite nanoparticles, which have the largest strain compared with halloysite and chrysotile, can still be composed of proto-imogolite (particles with n ≪ 1) if their concentration in the solution is below a certain threshold, according to Belloni and Thill (Reference Belloni, Thill, Yuan, Thill and Bergaya2016). Despite the fact that the model is focused on comparison of only two particles, it is related indirectly to the possible presence of a concentration threshold. Comparing energy curves at L 1 = 20 nm and L 1 = 30 nm (Fig. 4), two energy minima can be seen in the n < 1 and n > 1 areas divided by a barrier at n = 1. This means that the particle contained insufficient amounts of substance to form a scroll and it needed to gain it via recrystallization or oriented attachment with adjacent particles, which, in turn, depended on the parameters of the medium, including volume concentration. The n < 1 minimum itself is an additional hindrance to multi-walled nanoscroll formation.
The calculated results could be related to the experimental features in the preparation of the initial composition and to the hydrothermal treatment conditions needed to synthesize pure halloysite nanoscrolls. An increase in the σe value (Fig. 3) could avoid the platy particle growth demonstrated in Fig. 4 and could probably be achieved by varying the composition of the hydrothermal medium, as well as by dehydration of the initial composition by heat treatment, because (see discussion of Table 1) oxide surfaces have a larger σ than hydroxide surfaces. Dehydration should also decrease differences in σ on the opposite sides of the layer, which, in the case of halloysite, hinders scrolling. In addition, the components of the initial composition should be put in chemical contact, instead of using mechanical mixtures of Al2O3 and SiO2, because recrystallization under hydrothermal conditions is usually accompanied by hydration (Korytkova et al. Reference Korytkova, Maslov, Pivovarova, Drozdova and Gusarov2004, Reference Korytkova, Pivovarova, Semenova, Drozdova, Povinich and Gusarov2007; Ogorodova et al. Reference Ogorodova, Kiseleva, Korytkova and Gusarov2007).
Conclusions
The aim of the present study was to consider the question, from the perspective of energy modeling, “why do synthetic chrysotile layers form nanoscrolls with ease, whereas halloysite layers tend to form plates?” Analysis of the size mismatch between the sheets has shown that the halloysite layer had even greater scrolling potential than that of chrysotile (the radii of the mechanically unstressed layers were 3.3 nm and 8.8 nm, respectively). These two layers had opposite directions of scrolling, however, and the surface-energy difference on the opposite sides of the halloysite layers acted against the strain-energy component. This was the reason for the striking difference between the preferred size parameters of chrysotile (7–8 layers) and halloysite (21–22 layers) nanoscrolls. Moreover, the sufficiently low specific surface energy of the halloysite edge surfaces enabled the formation of a multi-layered plate instead of a nanoscroll. An halloysite particle ‘chose’ to be a plate or to scroll at the very beginning of the growth process, and changing the morphology later, in spite of the energy gap between plate and scroll states, was difficult.
ACKNOWLEDGMENTS
The research was supported by the Russian Science Foundation Grant 16-13-10252. The author thanks E.K. Khrapova, Dr T.P. Maslennikova, and Prof. V.V. Gusarov for fruitful discussions.
Compliance with Ethical Standards
Conflict of Interest
The corresponding author states that there is no conflict of interest.